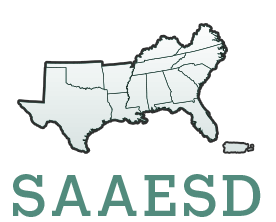
S1083: Ecological and genetic diversity of soilborne pathogens and indigenous microflora
(Multistate Research Project)
Status: Active
S1083: Ecological and genetic diversity of soilborne pathogens and indigenous microflora
Duration: 10/01/2023 to 09/30/2028
Administrative Advisor(s):
NIFA Reps:
Non-Technical Summary
Statement of Issues and Justification
Agricultural and horticultural crops are produced with an estimated market value of $241 billion in the United States for 2021 (USDA 2021). Soil-borne plant pathogens are diverse and encompass microorganisms such as fungi, oomycetes, bacteria, viruses, and nematodes that cause pre- and post-emergence damping-off, root and crown rots, vascular wilts, as well as foliar blight in these crops and amenity plantings. Soil-borne pathogens often survive for long periods on host plant residue, soil organic matter, or as free-living organisms. Soil-borne pathogens may have broad host ranges, and crop species may be susceptible to several different pathogens. Due to the menagerie of plant species produced by growers, and differences in the scales of production systems, soil-borne disease management is challenging. Interactions with soil texture, chemistry, and environmental conditions make soil-borne disease management challenging. In addition, temporal and spatial scales play a major role in understanding the role of the host, and environment on the pathogen effects. Diseases caused by these pathogens reduce plant performance; increase costs to the grower and cause potential ecological damage to the natural environment. In the US, of 2000 plant diseases on major crops more than half are caused by soilborne pathogens (Panth et al. 2020). As an example, losses due to soil-borne diseases in Georgia in 2014 were estimated to be $149 million in ornamental and turf production (Little, 2014).
In 2018, the National Integrated Pest Management (IPM) Road Map was released and two major focuses were: “Develop advanced management tactics for specific settings that prevent or avoid pest/disease attack” and efforts to “Improve the efficiency of suppression tactics and demonstrate least-cost options and pest/disease management alternatives” as critical research needs (Lane et al. 2023, IPM 2013). Soil-borne diseases are becoming more difficult to manage because of increased pathogen resistance and restrictions on the use of some chemicals. Conventionally, soil-borne diseases are controlled by using soil fumigants, in-furrow fungicides, or fungicide seed treatment. Once a widely used fumigant, methyl bromide, was phased out of use in 2005 due to its negative effect on the stratospheric ozone layer (Dungan et al., 2003). The loss of methyl bromide has promoted increased interest in alternative methods to control soil-borne diseases. Although safer or less environmentally impactful chemical and non-chemical plant disease management methods have been developed, their results are still inconsistent (Keinath et al., 2000) and less effective than the previous standard, methyl bromide (Gerik and Hanson, 2011).
Some soil-borne pathogens have broad host ranges, reducing the effectiveness of crop rotations in soil-borne disease management. Further, the susceptibility of plants to disease is related to their macro- and micronutrient status; both excesses and deficiencies in nutrients predispose plants to disease (Dick and McCoy, 1993; Maynard, 1994; Workneh and van Bruggen, 1994). Clearly, additional soil-borne disease management strategies suited to the practice of sustainable production are urgently needed. Novel methods of control include the combination cropping systems, including cover crops, sanitation such as anaerobic soil disinfestation, and biofumigants among others (Panth et al. 2020; Chellemi et al. 2016). However, a better understanding of how novel practices could modify the soil environment and increase the control of diseases is not well known.
Over the last 15 years, there have been surprising and exciting innovative discoveries for natural methods to suppress or eliminate plant pathogens, and/or protect crop plants. Intensive studies of disease-suppressive soils have led to the development of new methods of analysis (Gross et al., 2007; Borneman et al., 2007; Bolwerk et al., 2005; Benitez et al., 2007) and new insights into the nature of soil-borne disease suppression (Hoitink and Boehm, 1999; Han et al., 2000; Krause et al., 2003; Alfano et al., 2007). The active management of soil microbial communities can be an effective approach to developing natural suppression of diseases and improving crop productivity (Raaijmakers and Mazzola 2016; Mazzola 2004). This involves adjusting the types and timing of organic inputs, such as cover crops, animal manures, composts, compost teas, and crop sequencing. Such approaches have been shown to provide site-specific reductions in disease incidence (Abbasi et al., 2002; Rotenberg et al., 2005, Stone et al., 2003; Darby et al., 2006; Larkin et al.,2006; Larkin, 2008). This technology fits the general requirements of sustainable agriculture in that it utilizes natural means to control diseases. However, standardized and reliable techniques for pathogen suppression have not been developed and widely tested on different crop production systems for controlling soil-borne diseases. In part, this is due to the wide variety of organic amendments that are available and their variable effects depending on the chemical makeup of organic substrates, soil types, and/or local climatic conditions.
Soil incorporation of Brassica or other cover crops has the ability to suppress soil microorganisms through the hydrolysis of glucosinolates (GSL) into isothiocyanate, a natural biofumigant (Kirkegaard et al., 1993, Matthiessen and Kirkegaard, 2006). GSL content and concentration differs among Brassica cultivars, the development stage of the plant (Bellostas et al., 2007), and the end product formed by hydrolysis of the GSL, so different Brassica cultivars may have different levels of potential to control pathogens (Motisi et al., 2009). Therefore, it is important to study the different GSL-hydrolyzed end products produced by different Brassica crops and their effect on major soil-borne diseases. Cereal rye is one of the most prevalent cover crops used in the US since it is easier to adapt to different production systems (Kim et al. 2020; Wallander et al. 2021). However, like with Brassica and other crops, there is limited information on the mechanisms and how cultivars could modify their response in soil and management to soilborne pathogens, there are cases of actually being detrimental carrying pathogens (Bakker et al. 2016). Incorporation of biofumigant use in the crop production cycle may provide additional successful and sustainable solutions for improving soil quality and enhancing natural soil-borne disease control. The effective use of biofumigants in crop production appears to be limited by a range of factors, which needs to be examined to provide effective recommendations to growers.
Several commercially available biopesticides are composed of specific isolates of soil microorganisms that were selected for their capacity to suppress a range of pathogens. These biopesticides may operate through multiple mechanisms, such as niche exclusion, biocidal/biostatic effects, antibiosis, predation, and parasitism (Handelsman and Stabb, 1996; Fravel, 2005). Some of the most common microbe-based biopesticides contain bacterial isolates of Bacillus spp., Pseudomonas spp., or Streptomyces spp., or fungal isolates of Gliocladium spp. or Trichoderma spp. (Fravel, 2005). Many of these organisms suppress disease and associated pathogen populations through multiple mechanisms. Numerous studies and reviews have documented the potential of microbe-based biopesticides to suppress both foliar and root diseases (Doumbou et al., 2001; El-Tarabily and Sivasithamparam, 2006; Emmert and Handelsman, 1999; Fravel, 2005; Jacobsen et al., 2004). However, the general consensus among these reviews is that integration is the key to obtaining consistent activity from biopesticides (Fravel, 2005; Jacobsen et al., 2004). Amongst the microorganisms developed for the biological control of plant diseases, Bacillus spp. particularly have been exploited due to their stability for long periods of time and their antimicrobial activity (Fravel, 2005). Suppression has been attributed to the direct antagonism of pathogen growth through the production of various metabolic byproducts (Peypoux et al., 1999; Bonmatin et al., 2003). Additional work also demonstrated that some of these metabolites may also stimulate plant defenses, conferring an additional layer of control (Ongena et al., 2007).
Several species of Streptomyces have also been evaluated for disease control, mostly against fungal pathogens. A screen using the fermentative products from several Streptomyces spp. isolated from soil were found to inhibit several fungal pathogens, including Pyricularia grisea, Botrytis cinerea, Phytophthora infestans, Puccinia recondita, and Blumeria graminis (Park et al., 2003). Streptomyces spp. have also been evaluated as possible agents for the control of potato scab, caused by S. scabies (Liu et al., 1995). Several of these microorganisms have become commercial products, increasing the interest in the industry for biological agents owe(Olowe et al. 2020; Breakfield et al. 2021).
The adoption of biopesticides has increased. But there is a need for biopesticides to provide workable disease management solutions for growers. Demand for biopesticides has continued to expand dramatically in the last 15 years. However, despite substantial growth in the industry and markets, there is still a lack of publicly available data that substantiates efficacy and return on investment for most products. There is a critical need to develop and disseminate science-based informational resources that will promote useful and sustainable adoption by growers that experience significant plant disease pressure. Lack of knowledge about disease management and fears of extensive losses due to disease and other pests contribute to the lack of adoption of farming practices (Walz, 1999; Lotter, 2003). However, growers' specific knowledge gaps regarding disease management in agricultural crops are not well documented. It is critical that growers not only choose but also use biopesticides appropriately within a comprehensive disease management system or sound integrated pest management.
Given the need for sound integrated pest management, approaches coordinating chemical and biological controls are needed. Recently emphasis has been placed on understanding the phytobiome of plants or the soil microbiome. While metagenomic techniques, in theory, should allow for identification and association with soil-borne diseases, more importantly, these techniques offer the opportunity to understand biological suppressiveness (Weller et al., 2002). However, there are limitations to these methods (Bakker et al. 2012; Fierer 2017), so evidence must be combined with spatial analysis (Liu, Griffin, and Kirkpatrick, 2014) or analyzed across multiple locations and years to limit sampling error and bias (Paul et al., 2011). Recently, the use of indigenous vs. synthetic microbiomes to control soil-borne diseases was explored (Mazzola and Freilich, 2017). There are clear advantages with respect to survival and likely efficacy when microorganisms adapted to the specific environment or competing for a similar niche in the phytobiome are used. Numerous examples are presented in the literature (albeit determined with more traditional laboratory and field techniques). For example, fungi antagonistic to Rhizoctonia have been identified and have been shown to have the ability to reduce the severity of disease on numerous crops. Of these, some are other nonpathogenic Rhizoctonia solani, binucleate Rhizoctonia, or fungi in other genera such as Trichoderma spp. or a sterile white basidiomycete. Recently, R. solani AG11 was shown to be associated with reduced soybean seedling disease caused by Pythium spp. (Spurlock et al., 2016).
Ichielevich-Auster (1985) showed that a nonpathogenic isolate of R. solani AG4 reduced damping off in seedlings of cotton, radish, and wheat by R. solani and R. zea by 76-94%. Cardoso and Echandi (1987b) reported binucleate Rhizoctonia-protected bean seedlings from a virulent root rot-causing isolate of AG4. In another study (Cardoso and Echandi, 1987a), binucleate Rhizoctonia isolates protected snap bean seedlings from an isolate of AG4 causing root rot by what was deemed a metabolic mechanism of protection. Snap bean seedlings were exposed to the binucleate isolate and then replanted. Replanted seedlings maintained a level of suppression of the pathogen. Root exudates from the binucleate-treated seedlings were also inhibitory to the pathogen in vitro. Burpee and Goulty (1984) also reported disease suppression by binucleate Rhizoctonia on brown patch disease caused by R. solani AG2-2 III B on creeping bentgrass. Sumner and Bell (1994) reported a significant efficacy of a binucleate Rhizoctonia AG-2 and T. hamatum against R. solani AG4, and recently Spurlock (2009) found an unidentified sterile white basidiomycete that protected zoysiagrass from R. solani AG2-2.
New project members include researchers with diverse expertise that will work together to address the objectives set from different but complementary perspectives within the context of the phytobiome initiative and IPM, including mycology, microbiology, basic molecular biology, genetics, bioinformatics, population biology, evolutionary biology, metagenomics, metatranscriptomics, plant disease monitoring, spatial analysis, as well as traditional chemical and non-chemical control. The integrated effort of our research will lead to productive collaborations of relevance at the regional and national levels. The results obtained will be reported in peer-reviewed scientific journals, specialized disease management journals and online publications, and transferred to the broader community (such as growers, educators, academic, and industry collaborators) through extension education, college and graduate level courses, informational web pages, short video segments, and fact sheets.
The long-term goals of the proposed multi-state project are to investigate the impact of rhizosphere microbial communities on plant health and on the productivity of diverse cropping systems and to validate and evaluate different soil-borne disease management strategies under different environmental conditions.
Related, Current and Previous Work
Crop damage caused by soil-borne plant pathogens is the most yield-limiting factor when producing food, fiber, and ornamental crops (Weller et al., 2002). Around 2000 plant diseases n major crops more than half are caused by soilborne pathogens (Panth et al. 2020). Soilborne plant pathogens cause severe economic losses due to seed rots, pre- and post-emergence damping-off, pod rots, root and crown rots, vascular wilts, as well as foliar blight. Soilborne pathogens often survive for long periods on host plant debris, soil organic matter, or as free-living organisms (Agrios, 2005; Shurtleff and Averre, 1998). Each crop may be susceptible to several pathogens. Many factors including soil type, texture, pH, moisture, temperature, nutrient levels, and ecology affect the activity of soil-borne pathogens. In addition, the role of management plays a major role in how these pathogens and the associated microbial community change in order to manage the disease. The loss of methyl bromide has promoted increased interest in alternative methods to control soil-borne diseases. There is a big effort for research focused on sustainable practices such as cover crops and crop rotation systems and their role in soilborne pathogen control. Other practices like soil fumigants, in-furrow fungicides, or fungicide seed treatments are widely used to control soil-borne diseases.
A Southern Regional Project on soil-borne plant pathogens has been active since the initial project S-26 "The Relation of Soil Microorganisms to Soil-borne Plant Pathogens” was established in 1956. Throughout the series of projects, it has maintained active research on important soil-borne pathogens and examined the role of soil microflora in moderating the effects of these organisms. Other areas of emphasis during these projects have focused on the effects of the rhizosphere, the role of crop residues, amendments, and cultural practices on pathogens and other soil microflora. In 1995, the project on soil-borne pathogens started an emphasis on the introduction and evaluation of biocontrol agents across crops and environments and received funding for these evaluations. In project S-1028, the regional evaluation of biocontrol agents was extended to broccoli. Investigators in the project conducted research under the project on chemical and biological control of pathogens on summer squash (Seebold et al. 2008), tomato (Gwinn et al. 2010), cotton (Hu et al. 2011), snap bean (Canaday and Schmitthenner, 2010, Canaday, 2011), and soybean (Mengistu et al., 2011). Amendments of Monarda, brassicas, or legumes as green manure for disease control were examined in cotton, tomato, and watermelon and additional amendments were examined in the regional broccoli experiments and a variety of organic amendments for Christmas trees. Rhizosphere microflora characterization was conducted for turf, Christmas trees, strawberries, watermelon, and cotton as part of the studies (Njoroge et al., 2008). The diversity of Rhizoctonia solani, Sclerotinia sclerotiorum, Verticillium dahliae, Sclerotinia minor, and Phymatotrichopsis omnivora, and Pythium spp. was noted. A standardized protocol from the regional effort was developed for the recovery of Rhizoctonia using a toothpick baiting technique and selective media (Spurlock et al., 2011). In the S-1053 project, a team of scientists from the University of Arkansas, Mississippi State University, and Louisiana State University named a new disease of soybean prevalent in those three states taproot decline (TRD). Through phylogenetic analysis of isolates collected from Arkansas, Louisiana, and Mississippi, as well as other known species of Xylaria, it was determined that this fungus was previously undescribed. The causal agent of TRD is a member of the X. arbuscula species aggregate and recently, it has been designated as X. necrophora (Garcia-Aroca et al., 2021), which was partly due to the efforts of members of this group. Investigators from Tennessee and Oregon conducted research on chemical and biological control for controlling soil-borne diseases such as Phytophthora and Rhizoctonia with different application methods, intervals, and reduced-rate applications in ornamentals (Baysal-Gurel 2017a and b). Mycovirus epidemiology in R. solani on important row crops was examined and results indicate mycoviruses are commonly found in isolates of R. solani. More than 20 new viruses, belonging to at least three different virus families were discovered. As part of the S-1053 project, the genetic profiles of Pythium and Globisporangium isolates resistant and sensitive to mefenoxam were completed, the genetic diversity of three species of Globisporangium pathogenic on Douglas fir were examined and compared (Weiland et al., 2015). Monophyletic groups with high risk of developing mefenoxam resistance were identified within G. cryptoirregulare, as well as low-risk phylogenetic groups (Garrido et al., 2014). A draft of the genome of Sclerotinia minor was annotated and compared with the genomes of sister taxa (Espindola et al., 2015a). A bioinformatics tool for metatranscriptomic analysis for the detection of soil-borne fungi and oomycetes and functional analysis was developed (Espindola et al., 2015b). Investigators in Arkansas as part of S-1053 project showed that cotton seedling disease pressure varied spatially across the research field based on predictable soil factors. This information could be used for creating prescription maps for variable rate management. A multi-year, field study is being conducted in Texas to evaluate the impact of cover crops on various soil microbial populations in dryland cotton systems. The results indicate that cover crops have the potential to increase microbial biomass and mycorrhizal colonization of cotton grown under dryland conditions, especially early in the growing season, with potential benefits to cotton resilience and productivity.
More recently, project S-1083 has continued the work on fungicide resistance in soilborne pathogens, Gambhir et al. (2021) looked at Rhizoctonia zeae populations from corn and soybean using three chemistries, but most isolates were sensitive and combined fungicide resistance and further analysis included population genetics using markers derived from genome sequencing. With a set of markers, there was enough resolution to divide the population and the population is mixed, which could mean a higher risk for fungicide resistance. Taproot decline remains a focus of the project, combining remote sensing, disease severity, and molecular diagnostics to understand disease progression and epidemiology of the pathogen. Yield and plant stand was used as parameters, showing an effect of the cover crop without pathogen, but the effect increases with pathogen present. In 2021-2022, Fan et al. conducted a field trial focused on understanding the epidemiology of Taproot decline caused by X. necrophora. Soil and plant samples were collected for four physiological stages. Currently, samples are being processed for DNA extraction and using the qPCR developed for quantifying and evaluating the progression of X. necrophora under the three cultivars with high susceptibility to tolerant responses. Milestones for this project include developing an understanding of the biology and epidemiology of X. necrophora for improved disease management practices.
Cover crop. Investigation into tillage and cover crop impacts on fungi will continue in future years and be expanded to other crops and to also determine treatment impacts on root-associated bacteria and fungi using next-generation sequencing approaches. Currently, members of the group in Ohio are evaluating different rotation systems including corn-soy rotation, corn-rye-soybean, and corn-fallow-soybean-wheat-corn. Most of the analysis is focused on corn-rye-soybean rotation to develop an understanding of beneficial nematodes, carbon pools, and their interactions. Two years of fungal and bacterial diversity data were generated for a corn-soybean and cover crop rotation system, and a total of 160 samples were processed for amplicon data. In Tennessee, another team member will assess biofumigant cover crops (arugula, mustard, dwarf essex rape, etc.) for soil-borne diseases (Phythophthora and Rhizoctonia) and improved plant growth in woody ornamentals and will characterize the linkages between microbial community structure and soil-borne disease suppression in woody ornamental nursery systems that employ biofumigant cover crops.
In the period from 2018 to 2022, 56 peer-reviewed papers, 81 abstracts, 35 extension publications, and 7 dissertations related to S-1083 project objectives were published by group members. In addition, the project allowed us to train at least 6 postdocs, 13 graduate students, and over 10 undergrads. To consolidate the work done by the group and summarize recent efforts and also discuss the next step, members are working on a review publication entitled: "The next frontier in plant pathology: researching soilborne plant diseases across space and time". This publication should be submitted by the renewal of the project.
Objectives
-
Evaluate the biology and diversity of soil-borne pathogens, associated antagonistic microorganisms, and environmental conditions in the context of the whole-system phytobiome.
-
Using culturing, amplicon, metagenomics, and spatial/temporal methodologies to understand microbial community dynamics and their associations with soil physicochemical properties, environmental factors, and disease suppression.
-
Evaluate the efficacy of disease management strategies on the management of soilborne pathogens.
-
Translate knowledge during stakeholder meetings and train and mentor students and early career personnel involved in the participating programs on soilborne pathology and ecology.
Methods
Objective 1. Evaluate the biology and diversity of soil-borne pathogens, associated antagonistic microorganisms, and environmental conditions in the context of the whole-system phytobiome.
The team will conduct characterization, histological, species diversity and population biology studies of soil-borne plant pathogens (such as Phytophthora spp., Rhizoctonia spp., Fusarium spp., Xylaria sp., Verticillium dahliae etc.) and antagonistic microorganisms of multiple crops, including ornamentals, vegetables, and row crops, among others. Furthermore, new diagnostic and fingerprinting methods will be developed using PCR, qPCR, next-generation sequencing, and metagenomic approaches.
Task 1.1. Determination of the identity and distribution of root-infecting fungi causing seedling and root diseases. A survey for soilborne pathogens will be conducted using grid sampling or at least documenting locations using GIS information. Root samples will be collected and processed for isolation on rich media such as PDA or semi-selective media such as PDA amended with antibiotics and/or fungicides. Sampling will be done on the field and horticultural crops. Identification of select root-infecting pathogens will be accomplished using PCR and sequencing or multiplex qPCR based on availability. One of the focuses is Verticillium dahliae, which is a major pathogen in potatoes and it could move to weeds. There are efforts on characterizing VCG to identify populations moving from weeds and other hosts to economically important crops. Within this framework, researchers within the project are optimizing the primers for the detection of the two VCG groups: VCG4A and 4B, which are relevant for production systems in Pennsylvania.
Task 1.2. Determination of factors associated with incidence and severity of Taproot decline; a newly described disease of soybean. Over the past decade, a soybean disease that causes conspicuous interveinal chlorosis and necrosis of foliage has been predominantly observed throughout the Mississippi River Valley. The disease was recently named taproot decline, the causal agent confirmed as a fungus belonging to the genus Xylaria, and it was recently designated Xylaria necrophora (Allen et al., 2017, Garcia-Aroca et al., 2021). Taproot decline may result in plant death at any soybean growth stage. Plants that are killed or display symptoms during seedling and early vegetative stages often go unnoticed, as neighboring plants rapidly outgrow them in addition to lower canopy diseases masking the symptoms associated with taproot decline. When extracted from the soil profile, affected plants easily break at the soil line and exhibit a dry-rot, and excised roots appear blackened with tap and lateral root necrosis. Stems split longitudinally near the crown oftentimes contain white mycelial growth within the pith along with mild vascular staining. Reproductive structures or stroma that produce conidia, defined as “dead man’s fingers” are produced by the pathogen and are often observed emerging from crop debris near infected plants. The foliar symptoms are the result of root dysfunction and may be observed between the cotyledon (VC) and beginning maturity (R7) soybean growth stages. These symptoms are often confused with many other important root, crown and stem diseases as well as nutrient deficiencies. To date, taproot decline has been positively confirmed in Alabama, Arkansas, Louisiana, Mississippi, and southern Missouri. Although taproot decline may be observed every year, incidence and severity vary on an annual basis. Xylaria necrophora is a soil-borne fungus and causal organism of taproot decline of soybean, is undergoing characterization to determine optimal growth, virulence, and the infection process. Histological studies will broaden the understanding of the infection and disease cycle of this pathosystem. There will also be continuing spatial analysis of the disease in Arkansas, Mississippi, and Louisiana to determine the economic impact of the disease as well as soil edaphic factors associated with disease development.
Task 1.3. Characterization of antimicrobial bacteria. To obtain antagonistic bacteria from crop rhizospheres, soils showing disease suppressiveness will be collected from root systems associated with different soil-borne diseases. Bacteria will be isolated using various selective culture media. Antagonistic bacteria to major fungal and bacterial pathogens of plants will be selected using plate assays (Lu et al., 2002). Initial identification of bacterial genera will be performed as described by Schaad et al. (2001). In addition, bacterial isolation will be conducted from the fresh vegetable crops, which will be used for searching fresh food-associated Burkholderia spp.. To further characterize bacterial isolates, routine bacteriological analysis will be performed as described by Schaad et al. (2001), including morphology of bacterial colonies, growth on various media, and Gram staining. Biochemical and physiological analyses will be performed using the API kits (bioMérieux, Inc. Durham, NC) as described by the manufacturer. Molecular techniques, such as the 16S rRNA gene sequence analysis, will be conducted as described by Baker et al. (Baker et al., 2003). To characterize the genes dedicated to antagonism, mutagenesis of the antagonistic isolates of interest will be performed using an EZ::TN transposon system (Epicentre Technologies, Madison, WI). Plasmid rescue techniques will be used to clone the transposon-targeted genes from the resulting mutants deficient in antifungal activities as described by the manufacturer. Partial genes associated with antifungal activity will be sequenced. To clone the intact genes of interest, a fosmid library will be constructed using the Copy Control Fosmid Library Production Kit (Epicentre Technologies, Madison, WI) according to the kit manual. Screening the resultant library using the DNA fragments of the targeted genes, as probes will identify the fosmids that carry the intact genes. Complementation of mutant strains will be performed with the identified intact gene carried by appropriate expression vectors (Cardona and Valvano, 2005). Sequence analysis of the targeted genes will provide insights for predicting the possible products contributing to antagonism. The success of cloning the partial genes dedicated to antifungal activities of isolate MS14 demonstrates that the procedures described above work efficiently for obtaining the genes of interest from bacteria. To perform site-direct mutagenesis of the genes of interest, a standard procedure will be used as described previously (Gu et al., 2009b). In brief, the wild-type gene fragment will be disrupted by the insertion of a kanamycin cassette into its open reading frame as described previously (Lu et al., 2002). A 1.1-kb DNA fragment, which carries the nptII gene without a transcriptional terminator, will be obtained from plasmid pBSL15 (Alexeyev, 1995). The nptII cassette will be inserted into the appropriate site of the gene fragment, which will be transferred to pBR325 (Prentki et al., 1981). The marker exchange procedure (Gu et al., 2009a) will be employed to generate the corresponding mutant.
Objective 2. Using culturing, amplicon, metagenomics, and spatial/temporal methodologies to understand microbial community dynamics and their associations with soil physicochemical properties, environmental factors, and disease suppression.
Task 2.1. Spatial/temporal analysis from diversified cropping systems. Plant and soil sampling schemes will be designed to evaluate spatial variation within and between field sites, as well as within and between seasons (temporal variation and microbial succession). These diversity surveys, coupled with soil and agronomic data, will provide information on field management and soil conditions at which particular microbial taxa, or groups of taxa, either pathogenic or beneficial, are predominant. Further, research will be focused on microbial groups consistently showing responses across field sites and years, and will relate to the dynamics of core plant microbiome establishment. Rhizospheric and endophytic bacterial and fungal isolates will be used in order to monitor the dynamics of plant infection by different organisms. These experiments will be performed in greenhouse settings and microbial colonization will be measured through molecular approaches such as quantitative PCR. This work will likely include multiple crops across participating states. The exact locations and production systems have yet to be determined.
Task 2.2. Determination of the fungal community compositions using Illumina sequencing methods. Over the last decade next generation sequencing has become an important tool for conducting culture-independent surveys (Hibbet et al., 2011; Brown et al., 2013). A high percentage of the fungal sequences have never been identified or are unknowns since they have never been found in nature or are unculturable. Unfortunately, some of the species may be important or critical to understanding agricultural and forest microbial dynamics over time and the subsequence impacts of continuous cropping, artificial management, and natural inputs being added to cropland and forest health due to environmental changes such as nutrition. Therefore, in order to track changes in the pathogen and mycorrhizal communities associated with environmental causes or specific management practices with those ecosystems, whole-community (Illumina MiSeq) data is the most accurate approach for understanding microbiome fluctuations within farm sites and forest habitats. As an example, new peanut production areas in Mississippi currently have minimal soil-borne disease impacts, and baseline data from these sites will be used to monitor continuous cropping systems to determine the microbiome community spatiotemporal changes as they impact yields.
The team members propose to utilize soil samples collected for the measurement of nutrient levels with the same soil samples, extract fungal DNA from these samples, and determine the fungal community compositions using Illumina sequencing methods. To identify species, community composition, and abundance Illumina sequencing will be employed using primers concentration on ITS 2 gene region for sequencing. Potentially for each sample, Illumina sequencing can identify up to 1500 fungal species (Gihring et al., 2011). DNA will be extracted from these soil-litter samples using several protocols including Mo Bio Power® kits and CTAB as needed. The variable region ITS2 rDNA of fungi as stated above will be PCR amplified and analyzed using Illumina sequencing (Illumina Technology, Eurofin MWG). Each sequence will be assigned to its corresponding OTU (operational taxonomic unit) using the rDNA databases (RDP with 292,547 fungal ITS rDNA) for identity analysis. UNITE taxonomy reference database (http://unite.ut.ee/respository.php) will be specifically used to define specific taxa within OTU groupings. Finally, we will perform statistical analyses to examine the relationship between nutrient data and fungal community composition and species diversity measures. All statistical analyses will be carried out in R (version 4.1, Development Core Team, 2014) and used the Type 1 error rate of α = 0.05 after post-hoc statistical corrections when appropriate. Kruskal-Wallis rank sum tests and Dunn’s post-hoc corrections (posthoc.kruskal.dunn.test) will be carried out in the PMCMR package (Pohlert, 2016). Stepwise regression model selections were performed via the MASS package (Venables and Ripley, 2002). NMDS (metaMDS), perMANOVA (adonis), and environmental vector fitting (envfit) will be conducted in the vegan package (Oksanen et al., 2017) and the pairwise comparisons of sites using Bray-Curtis distances (pairwise.perm.manova) will be conducted in the RVAideMemoire package (Hervé, 2017).
Task 2.3. PCR amplification of the V2 region of 16S rDNA and Illumina sequencing. Bacterial DNA will be extracted using a CTAB protocol (Ausubel, 1988). The variable regions (V1-2) of bacterial 16S rDNA will be PCR amplified using the primers 27F and 338R (Ravel et al., 2011). The 338R primer includes a unique sequence tag to barcode each sample as described previously (Ravel et al., 2011). The primers are as follows: 27F-5’-GCCTTGCCAGCCCGC TCAGTCAGAGTTTGATCCTGGCTCAG-3’ and 338R-5’-GCCTCCCTCGCGCCATCAG NNNNNNNNCATGCTGCCTCCCGTAGGAGT-3’, where the underlined sequences are the sequencing primers in 27F and 338R, respectively, and the underlined letters denote the universal 16S rRNA primers 27F and 338R. The 8-bp barcode within primer 338R described by Ravel et al. ( 2011) will be adapted and is denoted by 8 Ns. To recover as many 16S rDNAs as possible, gradient PCR will be performed with annealing temperatures between 48-58°C (Sagaram et al., 2009). The PCR products will be analyzed using Illumina sequencing. The sequencing run generates more than 1,000,000 single reads with an average read length of 310 bases, which is good enough for the computation of bacterial richness and abundance. In order to reduce costs, six samples from three healthy plants and three diseased plants will be PCR amplified with the bar-coded reverse primers (Miller et al., 2009, Ravel et al., 2011). Equimolar amounts (100 ng) of the PCR amplicons from six samples will be mixed in a single tube. Pooled PCR products will be purified using a Promega PCR purification kit. Sequencing raw data will be trimmed and filtered using a customized Perl script to minimize the effects of poor sequence quality and sequencing errors by removing (i) barcoding and adapter sequences; (ii) sequences containing more than one ambiguous base call, (iii) sequences that are shorter than 300 nucleotides (excluding bar-coded primers); and (iv) the reads having less than 60% match to a previously determined 16S rRNA gene sequence. The whole data set will be divided into six subdata sets based on their bar codes, which will be used for further computation of bacterial richness and diversity. To assign each sequence to its corresponding OTU (operational taxonomic unit), sequence identity will be calculated as described previously (Turnbaugh et al., 2009). We will group the 16S rDNA region into OTU using a customized Perl script or other algorithms, such as Cd-Hit (Li & Godzik, 2006), with a sequence identity threshold of 97%, which is commonly used to define species-level phylotypes. The bacterial 16S rDNA database (Cole et al., 2009), will be used for identity analysis. To determine the abundance of individual OTUs in each sample, a customized Perl script will be written to identify the members of each sequence by performing a local BLAST similarity search against each of the trimmed subdata bases of the corresponding sample. Alternatively, we may adapt the methods described by Ravel et al. (2011). In that case, each processed 16S rRNA gene sequence read will be classified using the RDP Naïve Bayesian Classifier (Wang et al., 2007). This should result in almost all reads being assigned to a specific genus. Assignment at the species level for sequences mapping to a bacterial genus of interest will be accomplished by building a hidden Markov Model for each known species using the software HMMER and identifying the model with the highest score for each 16S rDNA sequencing read. Statistical analyses will be conducted on community clustering, diversity, and correlation as described by Ravel et al. (2011).
Objective 3. Evaluate the efficacy of soil-borne disease management strategies (chemical, biorational, biological, cultural) and characterize the associations among microbial community profile, soil physicochemical properties, environmental factors, and disease suppression.
The team will evaluate the efficacy of chemical, biorational/biological products; cultural approaches (cover crop/crop rotation, variety/cultivar screening etc.) for controlling soil-borne diseases caused by oomycetes (such as Phytophthora, Pythium) and fungi (such as Rhizoctonia) and characterize the associations between microbial community profile, soil physicochemical properties, environmental factors and soil-borne disease suppression of multiple crops.
For all our experiments, we will follow the following model for analyses: Response (disease severity / growth / biomass) = host (cultivar / genotype) X Treatment (cover crop / biofungicide / endophyte / control). Data collected will be analyzed using mixed models and regression following appropriate distributions based on response variable types.
Task 3.1. Evaluation of Chemical and biorational products in greenhouse and field conditions (Participants: All states). The monitoring of fungicide resistance and efficacy of products is relevant for soilborne pathogens. Members of the group will collect isolates from their respective systems and samples will be processed to establish a baseline sensitivity or shifts to existing fungicide baselines against different chemistries. The testing will be based on previous methods using amended media and high-throughput plate method (Noel et al. 2019). The results of these analyses will determine the existence and prevalence of resistant isolates in fields where chemical compounds are used. In Tennessee, another team member will evaluate the efficacy of chemical and biorational products for controlling soil-borne diseases with different application methods, intervals, and reduced-rate applications in woody ornamentals.
Task 3.2. Evaluation of Plant growth-promoting rhizobacteria (Participants: TN, OH). The use of microorganisms, such as plant growth-promoting rhizobacteria (PGPR), in sustainable productions is promising (Adesemoye et al., 2008; Raupach and Kloepper, 1998; Pal and Gardener, 2006) and has great potential in organic and conventional productions. There are many biological products currently available on the market which are based on PGPR. There is limited information on their efficacy against soil-borne pathogens such as Fusarium and Rhizoctonia species. Although there is substantial published literature on the effectiveness of PGPR conducted under laboratory and greenhouse environments but information on field applications is limited. More field studies as well as more greenhouse research will be conducted to better understand the factors that may affect the practical application of these biological control methods and how efficacy could be improved. Studies will examine possible mechanisms by PGPR, so that the knowledge from the studies could be leveraged to improve efficacy. Metabolite production is an important mechanism that will be studied. Bacillomycin, fengycin, iturin, surfactin, and difficidin, are examples of antifungal lipopeptides produced by PGPR (He et al., 2012; Bais, et al., 2004), which play roles in biological control. The practical applications of important metabolites in terms of their roles will be studied and findings will be helpful in developing sustainable management strategies for soil-borne pathogens.
Task 3.3 Evaluation of Variety/cultivar against prevalent and emergent soilborne pathogens (Participants: All states). Cultivar/variety screening for soil-borne pathogens including but not limited to Rhizoctonia spp. and Phytophthora spp. will be done in natural and artificially infested field/greenhouse environments. Field crops would be planted early season (prior to optimal planting dates) and irrigated to provide a conducive environment for disease development. Visual observation of disease and in-season/throughout experimental period evaluations will be recorded to determine the effects of pathogens on varieties/cultivars. Harvest/plant growth data will be used to determine losses/damage associated with disease. Pathogen isolations will be completed to confirm a particular microorganism has caused the observed symptoms.
Task 3.4. Characterization of endophytic bacteria associated with soybean resistance to charcoal rot disease. Endophytic bacteria of soybean root systems will be isolated and characterized using a routine culture-dependent method. When charcoal rot symptoms are visible, stems (10 grams, 5 cm above soil line) from the two types of plants (symptomatic or asymptomatic) will be collected and the composition and population of bacteria will be analyzed using routine methods as described by Schaad et al. (2001). Soybean root parts will be washed and surface sterilized using 70% alcohol and bleach (3% sodium hypochlorite) with Tween 20 (0.1%). Plant tissues will be broken into fine pieces and suspended in 10 ml of 0.01M phosphate buffer for 10 minutes. The resulting bacterial suspension will be serially diluted and spread onto different types of rich culture media, such as NBY for most bacteria (Vidaver, 1967) and NPPC for Actinomycetes (Williams and Davies, 1965). Sequencing the 16S rDNA of the isolates will be performed for bacterial identification using universal primers 27F and 1492R (Tanner et al., 2000) to confirm their identity. Symptomatic and asymptomatic plants (at least six of each type in each year) collected from diseased patches will be analyzed. All plants will be collected from the same soybean field to reduce possible variations caused by soil textures and culture conditions. Soybean stems will be collected and treated as stated above. Tissue homogenates will be fractionated by a series of differential centrifugations followed by a Nycodenz density gradient centrifugation (Ikeda et al., 2009). After a series of centrifugation, the whitish band located at the interface of the upper and lower phases will be collected as a bacterial cell fraction. The bacterial suspension (~500 µl) will be mixed with an equal volume of sterilized water in a 1.5-ml microtube and centrifuged. The resulting bacterial pellet will be used for the extraction of genomic DNA.
Task 3.5. Investigation of the effects of inoculation of endophytic bacteria on the fungal pathogen, disease reaction, and soybean growth. Plate bioassays will be used to evaluate direct interactions of the endophytic bacteria with the fungal pathogen M. phaseolina (Lu et al., 2005). The inhibitory zones of the bacteria on the fungal pathogens will be recorded to evaluate the direct effects on the pathogen growth. Soil with the pathogenic fungus M. phaseolina will be used as described previously (Su et al., 2001). The inoculum density (CFU per gram of inoculum mixture) will be determined by dilution plating. The soil will be infested with inoculum to yield 10 CFU/g of soil prior to filling pots. This level will be chosen because it represents an average level for M. phaseolina infestation in the soil at previous collections. To evaluate the roles of endophytic bacteria of interest, the soybean variety Asgrow 4651 will be used for these experiments in a greenhouse. Endophytic bacteria will be cultured to log phase and then washed twice using phosphate buffer. The selected bacteria will be used to inoculate the soils to reach a final concentration of 107 bacteria per gram of soil. Various combinations of endophytic bacteria of interest will be tested and appropriate controls (ex. phosphate buffer) will be included. Disease development will be recorded by using the rating scale described previously (Mengistu et al., 2007). Species-specific PCR primers will be developed based on the sequence data in NCBI, and the resulting primers will be used for the detection of the targeted bacteria and for quantification of the bacteria in plants. The population of the charcoal rot pathogen M. phaseolina also will be measured using quantitative real-time PCR with the specific primers of the pathogen and program described previously (Kishore et al., 2007). Correlation analysis between the fungal pathogen population, disease development, and the population of the bacteria will be performed. It is expected to find possible interaction relationships between these organisms.
Task 3.6. Evaluation of the impact of cover crops on soil health and survival of soilborne pathogens (Participants: OH, AR, TN, MS). Winter cover crops are increasingly being used by growers to control erosion and nutrient runoff from fields. Cereal rye is one of the most widely used cover crops available. It is easy to establish, produces large amounts of biomass that can suppress weeds, and timing of cover crop termination can give the grower some control of soil moisture at planting. Reductions in certain soybean diseases have been associated with cereal rye, particularly brown spot, sudden death syndrome, and soybean cyst nematode. The effect on seedling diseases is less clear. Cereal rye has been shown to reduce seedling disease caused by Rhizoctonia solani, but increase soil levels of pathogenic Pythium spp. and Fusarium spp. In addition, cereal rye may produce allelopathic compounds. We propose to conduct research with no-till cereal rye cover crop treatments: fallow, early termination, termination three weeks before planting, and termination at planting. Within each cover crop plot, we will have seed treatments with different active ingredients and/or cultivars to evaluate the responses. The soil parameters such as soil fertility (N levels), organic matter, electrical conductivity, and pH. The soil will also be analyzed for the density of major pathogen groups (fungi, bacteria, and oomycetes-Pythium spp.) and the diversity of soil microbial communities associated with the cover crops will be determined molecularly. Root-associated microbes will be monitored as described in objective 2.
Objective 4. Translate knowledge during stakeholder meetings and train and mentor students and early career personnel involved in the participating programs on soilborne pathology and ecology.
A strength of this project is our interaction with stakeholders. Our team participates and has strong interaction and communication with our stakeholders, with the goal of supporting agriculture by translating the knowledge generated during this project and implementing science- and risk-based management practices, improving food security, and developing sustainable practices. This allows us to expand our research work into approaches for outreach and education not only of stakeholders but also of early-career personnel and students participating in the project.
Measurement of Progress and Results
Outputs
- Understanding of the differences and similarities among particular agricultural and natural ecosystems regarding the genetic diversity of soil-borne pathogens.
- Improved control of soil-borne diseases of different crops through usage of cultural methods.
- Understanding of the interactions between fungi/oomycetes and bacteria present in soil and plant microbial populations.
- Understanding of the usefulness and feasibility of inoculation of plant endophytes for management of soil-borne diseases
- Optimized disease management protocols, using targeted management of field soil-borne diseases according to spatial distribution of inocula.
- Understanding of the impact of exposure to subinhibitory fungicides on the pathogenicity of soil-borne plant pathogens.
- Awareness of the available germplasm and the tolerance level to disease with and without chemical treatment.
- Improved protocols for collection, detection and diagnosis of soil-borne pathogens.
Outcomes or Projected Impacts
- Awareness of the diversity of soil-borne plant pathogens will allow effective disease management by taking into account the differences in sensitivity of each pathogenic species to particular chemical and biological treatments.
- Changes in soil-borne disease management recommendations with improved, efficacious, cost-effective and sustainable approaches will reduce plant losses, increase yields, and increase grower profits. This will lead to outcomes of growers changing their behavior to include more sustainable production practices and a reduction in the number of fungicides applied in agricultural production
- Understanding of plant-pathogen interactions with soil and plant microbial communities will result in identification of new biological control agents and management protocols
- Understanding the effects of subinhibitory effects of fungicides on diverse soil-borne plant pathogens will create awareness of the risks involved in the misuse of chemical control.
- Developing unique information on cover crop usage and innovative protocols by characterizing the impact of cover crops on soil-borne plant pathogenic populations and demonstrating the role of microbial diversity and ecology of the pathogenic genera in disease suppression.
- Improved protocols for detection and diagnosis of soil-borne plant pathogens will result in more effective management practices and higher crop productivity.
Milestones
(2023):- Review focused on emerging technologies and approaches to understand soilborne pathosystems - Collection, isolation, and identification of fungal and microbial strains interacting in each of the systems under study - Changes to extension publications will be recommended and manuscripts submitted to refereed journals. - Assessment of effects of subinhibitory fungicides on multiple fungal plant pathogens - Evaluation of current collection, detection and diagnosis protocols(2024):- Identification of microbial communities suppressive to soilborne plant pathogens - Evaluation of modified collection, detection and diagnostic protocols - Assessment of responses to fungicides in fungal plant pathogen populations - Data analyses, publication of scientific reports, and outreach materials - Progress reports at APS national and regional meetings as well as in the annual project meeting
(2025):- Identification of microbial species with potential for biological control of soilborne plant pathogens - Development of new collection, detection and diagnostic protocols - Assessment of the effect of management practices (i.e. fungicides) on fungal communities in the rhizosphere and phyllosphere - Updated management of Taproot decline in soybean - Data analyses, publication of scientific reports and outreach materials - Progress reports at APS national and regional meetings as well as in the annual project meeting
(2026):- Evaluation of microbial species with potential for biological control of soilborne plant pathogens - Validation of new collection, detection and diagnostic protocols - Assessment of the effect of management practices (i.e. fungicides, cover crops) on fungal communities in the rhizosphere and phyllosphere - Progress reports at APS national and regional meetings as well as in the annual project meeting - Development and delivery of extension education and outreach materials
(2027):- Completion of data analyses, publication of scientific reports and outreach materials - Extension education and outreach presentations and delivery of educational materials - New project: Updating issues and objectives for renewal
Projected Participation
View Appendix E: ParticipationOutreach Plan
Research results will be published in extension publications, non-refereed but peer-reviewed reports, and refereed journal articles to ensure access to research results by growers, the agricultural industry, other scientists, and interested parties. The results obtained and protocols developed will be reported in peer-reviewed scientific journals, specialized disease management journals, and online publications, and transferred to the broader community through extension education, college, and graduate level courses, informational web pages, and fact sheets.
Organization/Governance
This project has a very simple organization. There are two officers: the meeting chair and the meeting secretary. The meeting secretary becomes the meeting chair at the subsequent annual meeting. Thus, there is only one 'elected' position each year. The meeting secretary records the minutes of the annual meeting of the project membership and submits the minutes to the chairman within 30 days of the close of the meeting. The Chairman: (1) obtains approval for the annual meeting from the project administrative advisor (PAA), (2) prepares the annual meeting agenda, (3) notifies the membership and interested parties of the date, location, and time of the annual meeting at least 30 days in advance, (4) presides over the annual meeting, and (5) submits the annual report to the PAA within 60 days of the meeting.
There is one annual committee with one or more members - the local arrangements committee. Once the site (i.e., city and state) and date for the next annual meeting have been identified by the project membership attending the current meeting, this committee: (1) identifies the specific location for the annual meeting (building, room, time, etc.), (2) obtain approval for its use from the appropriate local authorities, (3) identifies local accommodations and reserves (if possible) a block of rooms for meeting attendees, and (4) identifies appropriate locations for meals, etc.
Other committees may be formed as identified by the needs of the membership, e.g. to facilitate the publication of project research results or to identify or formulate standard research procedures.
Literature Cited
Abbasi, P.A., Al-Dahmani, J., Sahin, F., Hoitink, H.A.J., Miller, S.A., 2002. Effect of compost amendments on disease severity and yield of tomato in organic and conventional production systems. Plant Disease 86: 156–161.
Adesemoye, A.O., Torbert, H.A., and Kloepper, J.W. 2008. Enhanced plant nutrient use efficiency with PGPR and AMF in an integrated nutrient management system. Canadian Journal of Microbiology 54:876-886.
Alexeyev, M. 1995. Three kanamycin resistance cassettes with different polylinkers. Biotechniques 18: 52-6.
Alfano, G., Lewis Ivey, M.L., Cakir, C., Bos, J.I.B., Miller, S.A., Madden, L.V., Kamoun, S. and Hoitink, H.A.J. 2007. Systemic modulation of gene expression in tomato by Trichoderma hamatum 382. Phytopathology 97:429-437.
Allen, T., Bluhm, B., Conner, K., Doyle, V., Price, T., Sikora, E., Singh, R., Spurlock, T., Tomaso-Peterson, M., Wilkerson, T. First Report of Taproot Decline, a Previously Undescribed Soybean Disease, in the Southern United States. 2017. Plant Health Progress. 18:35-40
Anasi, G.E. 2016. Evaluatin of fumonisin production in Fusarium proliferatum in response to subinhibitory doses of the fungicide iprodione. B.S. Microbiology thesis. Pontificia Universidad Catolica del Ecuador.
Ausubel, F.M. 1988. Current Protocols in Molecular Biology. New York: Greene Pub. Associates.
Bais, H. P., Fall, R., and Vivanco, J. M. 2004. Biocontrol of Bacillus subtilis against infection of Arabidopsis roots by Pseudomonas syringae is facilitated by biofilm formation and surfactin production. Plant Physiology 134: 307-319.
Baker, G.C., Smith, J.J., Cowan, D.A. 2003. Review and re-analysis of domain-specific 16S primers. J. Microbiol. Methods 55: 541-55.
Baysal-Gurel, F., Simmons, T., Kabir, Md.N., Liyanapathiranage, P. 2017a. Evaluation of biorational products and fungicides for the control of Phytophthora root rot of hydrangea, 2016. Plant Disease Management Report No. 11:OT004. Online publication. The American Phytopathological Society, St. Paul, MN.
Baysal-Gurel, F., Simmons, T., Liyanapathiranage, P., Kabir, Md.N. 2017b. Evaluation of biorational products and fungicides for the control of Rhizoctonia root rot of viburnum, 2016. Plant Disease Management Report No. 11:OT003. Online publication. The American Phytopathological Society, St. Paul, MN.
Bellostas, N., Sorensen, A.D., Sorensen, J.C., Sorensen, H. 2007. Genetic variation and metabolism of glucosinolates in cruciferous oilseed crops. In: Rapeseed Breeding: Advances in Botanical Research Academic Press/ Elsevier, Vol. 54.
Benítez, M.S., Baysal, F., Rotenberg, D., Kleinhenz, M. D., Cardina, J., Stinner, D., Miller, S.A., and McSpadden Gardener, B. 2007. Linking changes in bacterial populations with disease suppression, as affected by agricultural management strategies. Soil Biology and Biochemistry 39: 2289-2301.
Bolwerk, A., Lagopodi, A.L., Lugtenberg, B.J., Bloemberg, G.V. 2005. Visualization of interactions between a pathogenic and a beneficial Fusarium strain during biocontrol of tomato foot and root rot. Molecular Plant-Microbe Interactions 18: 710-721.
Bonmatin, J.M., Laprevote, O., Peypoux, F. 2003. Diversity among microbial cyclic lipopeptides: iturins and surfactins. Activity-structure relationships to design new bioactive agents. Comb Chem High T Scr 6: 541–556.
Borneman J. and Becker, J.O. 2007. Identifying microorganisms involved in specific pathogen suppression in soil. Phytopathology 45: 153-172.
Brown, S.P., Callaham, A.M., Oliver, A.K., and Jumpponen, A. 2013. Deep ion torrent sequencing identifies soil fungal community shifts after frequent prescribed fires in southeastern US forest ecosystem. FEMS Microbial Ecology 86: 557-566.
Burpee, L.L., and Goulty, L.G. 1984. Suppression of brown patch disease of creeping bentgrass by isolates of nonpathogenic Rhizoctonia spp. Phytopathology 74: 692-694.
Canaday, C.H. and Schmitthenner, A.F. 2010. Effects of chloride and ammonium salts on the incidence of Phytophthora root and stem rot of soybean. Plant Dis. 94: 758-765.
Canaday, C.H. 2011. Effects of seed treatment fungicides, biological agents, and potash fertilizers on snap bean seedling diseases, 2010. Plant Disease Management Reports 5: ST002.
Cardona, S.T., Valvano, M.A. 2005. An expression vector containing a rhamnose-inducible promoter provides tightly regulated gene expression in Burkholderia cenocepacia. Plasmid 54: 219-28.
Cardoso, J.E., and Echandi, E. 1987a. Biological control of Rhizoctonia root rot of snap bean with binucleate Rhizoctonia-like fungi. Plant Disease 71: 167-170.
Cardoso, J.E., and Echandi, E. 1987b. Nature of protection of bean seedlings from Rhizoctonia root rot by a binucleate Rhizoctonia-like fungus. Phytopathology 77: 1548-1551.
Cohen, M.J., Brown, M.T., and Shepherd, K.D. 2006. Estimating the environmental costs of soil erosion at multiple scales in Kenya using energy synthesis. Agriculture, Ecosystems and Environment, 114 : 249-269.
Cole, J.R., Wang, Q., Cardenas, E. 2009. The Ribosomal Database Project: improved alignments and new tools for rRNA analysis. Nucleic Acids Res 37: D141-D5.
Darby, H.M., A.G. Stone, and R.P. Dick. 2006. Compost and Manure Mediated Impacts on Soil-borne Pathogens and Soil Quality. Soil Sci. Soc. Am. J. 70: 347–358.
Dick, W.A., and McCoy, E.L. 1993. Enhancing soil fertility by addition of compost. In. Science and Engineering of Composting: Design, environmental, microbial and utilization aspects. Ed. H.A.J. Hoitink and H.M. Keener. Renaissance Publications, Worthington Ohio, pp. 662-644.
Doumbou, C.L., Hamby Salove, M.K., Crawford, D.L. and Beaulieu, C. 2001. Actinomycetes, promising tools to control plant diseases and to promote plant growth. Phytoprotection 82: 85–102.
Dungan, R.S., J. Gan, and S.R. Yates. 2003. Accelerated degradation of methyl isothiocyanate in soil. Water Air Soil Pollut. 142:299–310.
El-Tarabily, K.A. and Sivasithamparam, K. 2006. Non- streptomycete actinomycetes as biocontrol agents of soil-borne fungal plant pathogens and as plant growth promoters. Soil Biol Biochem 38: 1505–1520.
Emmert, E.A.B., Handelsman, J. 1999. Biocontrol of plant disease: a (Gram-) positive perspective. FEMS Microbiol. Lett., 171, pp. 1–9.
Espindola, A., Schneider, W.L., Melouk, H., Marek, S., Garzon, C.D. 2015. The draft genome of Sclerotinia minor. APS Annual Meeting. 778-P
Espindola, A.S., Schneider, W., Hoyt, P., Marek, S.M., Garzon, C.D.. 2015. A new approach for detecting fungal and oomycete plant pathogens in next generation sequencing metagenome data utilizing electronic probes. International Journal of Data Mining and Bioinformatics 12: 115-128.
Flores, F.J. and Garzón, C.D. 2013. Detection and assessment of chemical hormesis on the radial growth in vitro of oomycetes and fungal plant pathogens. Dose-Response. 11: 361-373.
Fravel, D.R. 2005. Commercialization and Implementation of Biocintrol1. Annu. Rev. Phytopathol. 43: 337–359
Garrido, P.A., Weiland, J.E., Flores, F.J., Herrero, M., Moorman, G.W., Daughtrey, M.L., Levesque, A., Garzon, C.D. 2014. Multilocus phylogeny of the Pythium irregulare complex. Phytopathology 104: 536-P.
Gerik, J. S. and Hanson, B.D. 2011. Drip application of methyl bromide alternative chemicals for control of soil-borne pathogens and weeds. Pest Management Science, 67: 1129– 1133.
Gihring, T.M., Green, S.J. and Schadt, C.W. 2011. Massively parallel rRNA gene sequencing exacerbates the potential for biased community diversity comparisons due to variable library sizes. Environmental Microbiology 14: 285-290.
Gross, H., Stockwell, V.O., Henkels, M.D., Nowak-Thompson, B., Loper, J.E., Gerwick, W.H. 2007. The genomisotopic approach: A systematic method to isolate products of orphan biosynthetic gene clusters. Chemistry & Biology (Cambridge) 14: 53-63.
Gu, G., Smith, L., Wang, N., Wang, H., Lu, S-E. 2009a. Biosynthesis of an antifungal oligopeptide in Burkholderia contaminans strain MS14. Biochem. Biophys. Res. Commun 380: 328-32.
Gu, G., Wang, N., Chaney, N., Smith, L., Lu, S.E. 2009b. AmbR1 is a key transcriptional regulator for production of antifungal activity of Burkholderia contaminans strain MS14. FEMS Microbiol. Lett 297: 54-60.
Gwinn, K.D., Ownley, B.H., Greene, S.E., Clark, M.M., Taylor, C.L., Springfield, T.N., Trently, D.J., Green, J.F., Reed, A., and Hamilton, S.L. 2010. Role of essential oils in control of Rhizoctonia damping-off in tomato with bioactive Monarda herbage. Phytopathology 100: 493-501.
Han, D.Y., Coplin, D.L., Bauer, W.D., and Hoitink, H.A. J. 2000. A rapid bioassay for screening rhizosphere microorganisms for their ability to induce systemic resistance. Phytopathology 90: 327-332.
Handelsman J., Stabb E.V. Biocontrol of soil-borne plant pathogens. Plant Cell.: 1855–1869.
He, P., Haoa, K., Blomc, J., Rückertc, C., Vater, J., Mao, Z., Wu, Y., Hou, M., He, P., He, Y., Borriss, R. 2012. Genome sequence of the plant growth promoting strain Bacillus amyloliquefaciens subsp. plantarum B9601-Y2 and expression of mersacidin and other secondary metabolites. Journal of Biotechnology 164: 281– 291
Hervé, M. 2017. RVAideMemoire: diverse basic statistical and graphical functions. R package. https://CRAN.R-project.org/package=RVAideMemoire. Accessed 1 March 2017.
Hibbett, D.S., Ohman, A., Glotzer, D., Nuhn, M., Kirk, P., Nilsson, R.H. 2011. Progress in molecular and morphological taxon discovery in fungi and options for formal classification of environmental sequences. Fungal Biology Reviews 25:38-47.
Hoitink, H.A.J. and Boehm, M.J. 1999. Biocontrol within the context of soil microbial communities: A substrate-dependent phenomenon. Annu. Rev. Phytopathol. 37: 427-446.
Hu, P., Wang, A.S., Engledow, A.S., Hollister, E.B., Rothlisberger, K.L., Matocha, J.E., Zuberer, D.A., Provin, T.L., Hons, F.M., and Gentry, T.J. 2011. Inhibition of the germination and growth of Phymatotrichopsis omnivora (Cotton Root Rot) by oilseed meals and isothiocyanates. Appl. Soil Ecol. 49: 68-75.
Ichielevich-Auster, M., Sneh, B., Koltin, Y. and Barash, I. 1985. Suppression of damping-off caused by Rhizoctonia species by a nonpathogenic isolate Rhizoctonia solani. Phytopathology 75: 1080-1084.
Ikeda, S., Kaneko, T., Okubo, T. 2009. Development of a bacterial cell enrichment method and its application to the community analysis in soybean stems. Microbial. Ecol. 58, 703-14.
IPM. 2013. National road map for Integrated Pest Management. http://www.ipmcenters.org/IPMRoadMap.pdf
Jacobsen, B.J., Zidack, N.K., Larson, B.J. 2004. The role of Bacillus-based biological control agents in integrated pest management systems: plant diseases. Phytopathology 94: 1272–1275.
Keinath, A.P., Batson, W.E., Jr. 2000. Evaluation of biological and chemical seed treatments to improve stand of snap bean across the southern U. S. Crop Prot. 19: 501-509.
Kirkegaard, J.A., Gardner, P.A., Desmarchelier, J.M., Angus, J. F. 1993. Biofumigation – using Brassica species to control pests and diseases in horticulture and agriculture. In: 9th Australian Research Assembly on Brassicas Eds. N Wratten and R J Mailer. pp 77–82. Agricultural Research Institute, Wagga.
Kishore, B.B., Saxena, A.K., Srivastava, A.K., Arora, D.K. 2007. Identification and detection of Macrophomina phaseolina by using species-specific oligonucleotide primers and probe. Mycologia 99, 797-803.
Krause, M. S., De Ceuster, T. J. J., Tiquia, S. M., Michel, F. C., Jr., Madden, L. V., and Hoitink, H. A. J. 2003. Isolation and characterization of rhizobacteria from composts that suppress the severity of bacterial leaf spot of radish. Phytopathology 93: 1292-1300.
Larkin, D., Vivian-Smith, G., and Zedler. J. B. 2006. Topographic heterogeneity theory and ecological restoration. In: D. A. Falk, M. A. Palmer, and J. B. Zedler, editors, pp 142–164. Foundations of restoration ecology. Island Press, Washington, D.C.
Larkin, R.P. 2008. Relative effects of biological amendments and crop rotations on soil microbial communities and soil-borne diseases of potato. Soil Biol. Biochem 40:1341-1351.
Li, W.Z., Godzik, A. 2006. Cd-hit: a fast program for clustering and comparing large sets of protein or nucleotide sequences. Bioinformatics 22: 1658-1659.
Little, E. 2014. http://extension.uga.edu/publications/files/pdf/AP%20102-7_2.PDF
Liu, D., Anderson, N.A. and Kinkel, L.L. 1995. Biological control of potato scab in the field with antagonistic Streptomyces scabies. Phytopathology 85, 827–831.
Liu, Z., Griffin, T., and Kirkpatrick, T.L. 2014. Statistical and Economic Techniques for Site-specific Nematode Management. Journal of Nematology 46:12-17.
Lotter, D. 2003. Organic agriculture. Journal of Sustainable Agriculture, 21: 59–128.
Lu, S-E, Scholz-Schroeder, B.K., Gross, D.C. 2002. Characterization of the salA, syrF, and syrG regulatory genes located at the right border of the syringomycin gene cluster of Pseudomonas syringae pv. syringae. Mol. Plant-Microbe Interact 15: 43-53.
Lu, S-E, Woolfolk, S., Caceres, J. 2005. Isolation and identification and genetic analysis of rhizobacteria antagonistic to plant soil-borne fungal pathogens. Phytopathology 95: 62-63.
Matthiessen, J.N. and Kirkegaard, J.A. 2006. Biofumigation and enhanced biodegradation: opportunity and challenge in soil borne pest and disease management. Critical Reviews in Plant Sciences, 25: 235-265.
Maynard, A.A. 1994. Sustained vegetable production for three years using composted animal manures. Compost Sci. Util. 2: 88-96.
Mazzola, M. 2004. Assessment and management of soil microbial community structure for disease suppression. Annu. Rev. Phytopathol. 42: 35-59.
Mazzola, M., and Freilich, S. 2017. Prospects for biological soil-borne disease control: application of indigenous versus synthetic microbiomes. Phytopathology 107: 256-263.
Medvecky, B.A., Ketterings, Q.M., and Nelson, E.B. 2007. Relationships among soil-borne bean seedling diseases, Lablab purpureus L. and maize stover residue management, bean insect pests, and soil characteristics in Trans Nzoia district, Kenya. App. Soil Ecol. 35: 107-119.
Mengistu, A., Ray, J.D., Smith, J.R., Paris, R.L. 2007. Charcoal rot disease assessment of soybean genotypes using a colony-forming unit index. Crop Sci. 47: 2453-2461.
Mengistu, A., Arelli, P.A., Bond, J.P., Shannon, G.J., Wrather, A.J., Rupe, J.B., Chen, P., Little, C.R., Canaday, C.H., Newman, M.A., and Pantalone, V.R. 2011. Evaluation of soybean genotypes for resistance to charcoal rot. Online. Plant Health Progress doi:10.1094/PHP-2010-0926-01-RS.
Miller, S.R., Strong, A.L., Jones, K.L., Ungerer, M.C. 2009. Bar-coded pyrosequencing reveals shared bacterial community properties along the temperature gradients of two alkaline hot springs in yellowstone national park. Appl. Environ. Microbiol. 75: 4565-4572.
Moncrief, I.R., Garzon, C.D., Marek, S.M., Stack, J.P., Gamliel, A., Garrido, P., Proaño, M.F., Gard, M., Dehne, H., Fletcher, J. 2016. Development of simple sequence repeat (SSR) markers for discrimination among isolates of Fusarium proliferatum. Journal of Microbiological Methods. 126: 12-17.
Motisi, N., Montfort, F., Dore, T., Romillac, N., Lucas, P. 2009. Duration of control of two soil-borne pathogens following incorporation of above- and below-ground residues of Brassica juncea into soil. Plant Pathology. 58: 470-478.
Nesme, J., Achouak, W., Agathos, S.N., Bailey, M., Baldrian, P., Brunel, D., Frostegård, Å., Heulin, T., Jansson, J.K., Jurkevitch, E., Kruus, K.L., Kowalchuk, G.A., Lagares, A., Lappin-Scott, H. M., Lemanceau, P., Le Paslier, D., Mandic-Mulec, I., Murrell, J.C., Myrold, D.D., Nalin, R., Nannipieri, P., Neufeld, J.D., O'Gara, F., Parnell, J.J., Pühler, A., Pylro, V., Ramos, J. L., Roesch, L.F., Schloter, M., Schleper, C., Sczyrba, A., Sessitsch, A., Sjöling, S., Sørensen, J. ,Sørensen, S.J., Tebbe, C.C. ,Topp, E., Tsiamis, G., J. van Elsas, D., van Keulen, G., Widmer, F.,Wagner, M., Zhang, T., Zhang, X., Zhao, L., Zhu, Y.G., Vogel, T.M., and Simonet, P. 2016. Back to the Future of Soil Metagenomics. Front Microbiol 7:73. doi: 10.3389/fmicb.2016.00073.
Njoroge, S.M.C., Riley, M.B., and Keinath, A.P. 2008. Effect of incorporation of Brassica spp. residues on population densities of soil-borne microorganisms and on damping-off and Fusarium wilt of watermelon. Plant Disease 92: 287-294.
Oksanen, J., Blanchet, F.G., Friendly, M., Kindt, R., Legendre, P., McGlinn, D., Minchin, P.R., O’Hara, R.B., Simpson, G.L., Solymos, P., Stevens, M.H.H., Szoecs, E., Wagner, H. 2017. Package vegan. R package. https://CRAN.R-project.org/package=vegan.
Ongena, M., Jourdan, E., Adam, A. Thonnart, P. 2007. Surfactin and fengycin lipopeptides of Bacillus subtilis as elicitors of induced systemic resistance in plants. Environ Microbiol 9: 1084–1090.
Pal, K.K., and Gardener, B.M. 2006. Biological control of plant pathogens. The Plant Health Instructor DOI: 10.1094/PHI-A-2006-1117-02.
Park, J.H., Choi,G.J., Lee, S.W., Jang, K.S., Choi,Y.H.C., et al. 2003. Screening for antifungal endophytic fungi against six plant pathogenic fungi. Mycobiology 31: 179–182.
Paul, P.A., L. V. Madden, C.A. Bradley, A.E. Robertson, G.P. Munkvold, G. Shaner, K.A. Wise, D.K. Malvick, T.W. Allen, A. Grybauskas, P. Vincelli, and P. Esker. 2011. Meta-analysis of yield response of hybrid field corn to foliar fungicides in the U.S. Corn Belt. Phytopathology 101:1122-32. doi: 10.1094/PHYTO-03-11-0091.
Peypoux, F., Bonmatin, J.M., Wallach, J. 1999. Recent trends in the biochemistry of surfactin. Appl Microbiol Biot 51: 553–563.
Pohlert, T. 2016. PMCMR: Calculate pairwise multiple comparisons of mean rank sums. R package. https://CRAN.R-project.org/package=PMCMR.
Pradhan, S., Flores, F.F., Walker, N.R., Melouk, H., Molineros, J.E. and Garzon, C.D. 2017. Chapter 10. Chemical Hormesis on Plant Pathogenic Fungi and Oomycetes. In: S. Duke,
- Kudsk, and K. Solomon Eds. Pesticide Dose: Effects on the Environment and Target and Non-Target Organisms. ACS Books. Chapter DOI:10.1021/bk-2017-1249.ch009.
Pradhan, S., Flores, F., Molineros, J.E., Melouk, H., Walker, N.R., and Garzon, C.D. 2016. Improved assessment of mycelial growth stimulation by low doses of mefenoxam in plant pathogenic Globisporangium species. European Journal of Plant Pathology. DOI 10.1007/s10658-016-1016-5.
Prentki, P., Karch, F., Iida, S., Meyer, J. 1981. The plasmid cloning vector pBR325 contains a 482 base-pair-long inverted duplication. Gene 14, 289-99.
Rapauch, G.S. and Kloepper, J.W. 1998. Mixture of plant growth-promoting rhizobacteria enhance biological control of multiple cucumber pathogens. Phytopathology 88: 1158-1164.
Raaijmakers, J. M., and Mazzola, M. 2016. Soil immune responses. Science. 352:1392–1393.
Ravel, J., Gajer, P., Abdo, Z. 2011. Vaginal microbiome of reproductive-age women. Proc. Natl. Acad. Sci. U. S. A. 108 Suppl 1: 4680-4687.
Rotenberg, D., Cooperband, L., Stone, A. 2005. Dynamic relationships between soil properties and foliar disease as affected by annual additions of organic amendment to a sandy-soil vegetable production system. Soil Biology & Biochemistry 37: 1343-1357.
Sagaram, U.S., Deangelis, K.M., Trivedi, P., Andersen, G.L., Lu, S.E., Wang, N. 2009. Bacterial diversity analysis of Huanglongbing pathogen-infected citrus, using PhyloChip arrays and 16S rRNA gene clone library sequencing. Appl. Environ. Microbiol 75, 1566-74.
Schaad, N.W., Jones, J.B., Chun, W. 2001. Laboratory Guide for Identification of Plant Pathogenic Bacteria. American Phytopathological Society. St Paul, MN.
Seebold, K.W., Holdcroft, A.M., and Dixon, E. 2008. Effect of potassium phosphite and fungicides on Phytophthora crown and fruit rot of summer squash, 2007. Plant Disease Management Reports 2:V005.
Spurlock, T.N., and Milus, E.A. 2009. Master’s thesis. University of Arkansas.
Spurlock, T., Rothrock, C., and Monfort, W. 2011. A new selective medium for isolation of Rhizoctonia spp. from soil. Phytopathology 101: S170.
Spurlock, T.N., Rothrock, C.S., Monfort, W.S., and Griffin, T.W. 2016. The distribution and colonization of soybean by Rhizoctonia solani AG11 in fields rotated with rice. Soil Biology & Biochemistry 94: 29-36.
Stone, A.G., Vallad, G.E., Cooperband, L.R., Rotenberg, D., Darby, H.R., James, R.V., Stevenson, W.R., Goodman, R.M., 2003. Effect of organic amendments on soil-borne and foliar diseases in field-grown snap bean and cucumber. Plant Disease 87: 1037–1042.
Su, G., Suh, S.O., Schneider, R.W., Russin, J.S. 2001. Host specialization in the charcoal rot fungus, Macrophomina phaseolina. Phytopathology 91: 120-6.
Sumner, D. R., and Bell, D.K. 1994. Survival of Rhizoctonia spp. and root diseases in a rotation of corn, snap bean, and peanut in microplots. Phytopathology 84: 113-118.
Tanner, M.A., Everett, C.L., Youvan, D.C. 2000. Molecular phylogenetic evidence for noninvasive zoonotic transmission of Staphylococcus intermedius from a canine pet to a human. J. Clin. Microbiol 38: 1628-31.
Turnbaugh, P.J., Hamady, M., Yatsunenko, T. 2009. A core gut microbiome in obese and lean twins. Nature 457: 480-4U7.
USDA. 2014. 2012 Census of Agriculture. Washington, DC http://www.agcensus.usda.gov/
Venables, W.N., Ripley, B.D. 2002. Modern Applied Statistics. Springer, New York.
Vidaver, A.K. 1967. Synthetic and complex media for the rapid detection of fluorescence of phytopathogenic pseudomonads: Effect of the carbon source. Appl. Microbiol 15: 1523-1524.
Walz, E. 1999. Final Results of the Third Biennial National Organic Farmers’ Survey. Organic Farming Research Foundation. Santa Cruz, California.
Wang, Q., Garrity, G.M., Tiedje, J.M., Cole, J.R. 2007. Naive Bayesian classifier for rapid assignment of rRNA sequences into the new bacterial taxonomy. Appl. Environ. Microbiol. 73: 5261-5267.
Weiland, J.E., Garrido, P.A., Kamvar, Z.N., Espindola, A.S., Marek, S.M., Grünwald, N.J., and Garzón, C.D. 2015. Population structure of Pythium irregulare, P. sylvaticum, and P. ultimum in forest nursery soils of Oregon and Washington. Phytopathology 105:684-694.
Weller, D.M., Raaijmakers, J.M., Gardener, B.B., and Thomashow, L.S. 2002. Microbial populations responsible for specific soil suppressiveness to plant pathogens. Annu Rev Phytopathol 40: 309-48. doi: 10.1146/annurev.phyto.40.030402.110010.
Williams, S.T., Davies, F.L. 1965. Use of antibiotics for selective isolation and enumeration of actinomycetes in soil. Journal of General Microbiology 38: 251-262.
Workneh, F., and van Bruggen, A.H.C. 1994. Suppression of corky root of tomatoes in organically managed soil associated with soil microbial activity and nitrogen status of soil and tomato tissue. Phytopathology 81: 688- 694.
Wu, T., Chellemi, D.O., Martin, K.J., Graham, J.H., Rosskopf, E.N., 2007. Discriminating the effects of agricultural land management practices on soil fungal communities. Soil Biology and Biochemistry 39: 1139–1155.