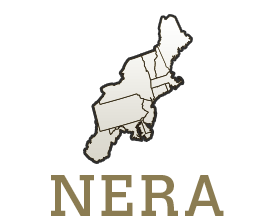
NE1013: Mechanisms of Plant Responses to Ozone in the Northeastern US
(Multistate Research Project)
Status: Inactive/Terminating
NE1013: Mechanisms of Plant Responses to Ozone in the Northeastern US
Duration: 10/01/2002 to 09/30/2007
Administrative Advisor(s):
NIFA Reps:
Non-Technical Summary
Statement of Issues and Justification
Surface level (tropospheric) ozone (O3) is all-pervasive and is considered to be the most important phytotoxic air pollutant across many parts of the US and rest of the world (Krupa et al. 2001). This O3 is also a "greenhouse or atmosphere warming gas", a component in the observed and/or predicted global climate change. Despite the national air quality regulations aimed at controlling surface level O3 pollution, it continues to be of major concern for crop production and forest health in the northeastern US and elsewhere (US EPA 1996). In the northeast, during 1996-2001, there were as many as 8 yearly violations of the US EPA's one-hour National Ambient Air Quality standard for O3 of 120 ppb. Although that standard has been revised to an average 8-hour 80 ppb value, the one-hour standard is still in place for monitoring non-attainment areas. In the application of the one-hour standard, based on the influence of meteorology in the production of O3, the second daily maximum hourly O3 value is used as an indicator of air quality attainment versus non-attainment and in examining the multi-year trends. The range of second maximum hourly concentrations in Pennsylvania, Massachusetts, New Jersey, New York and Maryland were respectively 78-129, 78-136, 80-137, 81-139 and 107-144 ppb. California long considered to be the area in the US with the most smog (includes O3) problems, exhibited a second highest maximum of 74-144 ppb, with a minimum value lower than Maryland (74 versus 107 ppb). Thus, the northeast clearly represents a geographic region of concern regarding O3 pollution. In comparison, the ranges of second maximum hourly values in the other participating states, Oregon, Virginia, Alabama, North Carolina and Minnesota were 70-136, 93-130, 84-118, 87-114 and 74-93 ppb (http://oaspub.epa.gov/airsdata/adaqs.trends?geo=01&cnty=&geoin). Ozone sensitive crops in the northeast and in other participating states include: alfalfa (Medicago sativa), bean (Phaseolus vulgaris), corn (Zea mays), cotton (Gossypium hirsutum and G. barbadense), grape (Vitis vinifera), potato (Solanum tuberosum), soybean (Glycine max), tobacco (Nicotiana tabacum), wheat (Triticum aestivum) and melon (e.g., Cucumis melo and Citrullus lanata). Among the 80 major tree species in the eastern US, black cherry (Prunus serotina), eastern white pine (Pinus strobus), green ash (Fraxinus pennsylvanica), sassafras (Sassafras albidum), sweetgum (Liquidambar styraciflua), quaking aspen (Populus tremuloides), willow (Salix spp.) and yellow or tulip poplar (Liriodendron tulipifera), are all known to be O3 sensitive (Krupa et al. 1998). In addition to causing visible foliar injury on sensitive plant species as reported from some 38 countries across the world, chronic exposures to O3 can result in reductions in crop yield and quality and in forest growth and productivity (Krupa et al. 2001). For example, ambient O3 exposures in Long Island, New York, in North Carolina and in California can be high enough to cause respectively a 25%, 39% and >50% biomass reduction in sensitive versus tolerant clones of white clover (Trifolium repens) (McGrath 2000; Heagle et al. 1995). Similarly, on Long Island, New York, a 21% yield reduction was observed in sensitive versus tolerant cultivars of snap bean (Phaseolus vulgaris) (McGrath 2000). Nutritive quality of bahiagrass (Paspalum notatum) and sericea lespedeza (Lespedeza cuneata) was decreased by 6% and 7%, respectively, as a result of chronic exposure to O3 (Powell et al. 1999; Muntifering et al. 2000). Independent of these limited efforts, there is need for a much broader conduct of these types of studies to assess O3 impacts at the regional (e.g., NE) and national level to validate crop loss estimates. During 1996, the US EPA estimated that O3 alone causes > $1 billion in crop loss annually. Similar estimates are not available at the present time for forests and native vegetation, although the adverse effects of O3 on our national parks and forests are evident (McLaughlin and Percy 1999). For example, in Acadia National Park in Maine, black cherry, quaking aspen, white ash (Fraxinus americana), jack pine (Pinus banksiana), big-leaf aster (Aster macrophyllus) and spreading dogbane (Apocynum androsaemifolium) are sensitive, exhibiting foliar injury (Kohut et al. 1997). Similarly, widespread O3-induced foliar injury was observed on the native black cherry and tall milkweed (Asclepias exaltata) in the Great Smoky Mountains National Park (Chappelka et al. 1997, 1999). Foliar injury on black cherry was also widespread in Pennsylvania and many other eastern states (Skelly et al. 1997). An intriguing aspect of O3 is its mechanism of action. Ozone is highly reactive, unstable and does not accumulate in plant tissue. Ozone is an oxidant, thus it can serve as an excellent model in understanding the mechanisms of action of oxidative stress caused among others, by biotic pathogens, herbicides such as paraquat, drought and increased ultraviolet (UV)-B radiation (part of global climate change) (Sandermann 1996). It is important to note that oxidative stress also occurs in humans. Based on the current state of our knowledge, there are many issues that clearly require significant attention. They include, (1) spatial and temporal distribution of O3-induced adverse effects on growth and productivity of crops and forests in the northeast and elsewhere in the US; (2) comparison of those results to other temperate regions such as Canada and the European countries; (3) mechanisms of O3 toxicity and tolerance in plants; (4) interactive effects of O3 with other growth regulating factors such as increases in carbon dioxide or changes in climatic factors (climate change) and incidence of pathogens and pests; (5) scaling the patterns of seedling responses to mature trees and forests; (6) effects of O3 in altering biological diversity and plant community structure within the context of climate change and (7) establishing biologically meaningful numerical relationships between ambient O3 and adverse plant responses. No single institution can fulfill the stated needs nor function in isolation, because many of the components from molecular to whole plant responses are inter-related. Addressing these issues has direct relevance to air quality regulatory policies and protection of the food supply and natural resources. Target audiences include, the US EPA, US National Park Service and USDA Forest Service, crop growers, members of the horticulture and forest production/management sectors, urban planners and industries contributing to the air emissions of O3 precursors. At the local level, audiences include: home and Master Gardeners, landscape and nursery managers, arborists and managers of public parks and recreational lands and individual crop producers. However, the underlying effort requires multi-disciplinary input and collaboration. With regard to the effects of O3 on plants, the NE-176 Multi-state Project represents the single largest cooperative work among the academic institutions and their scientists in the northeast and the US. In addition to experiment stations, program scientists include those from governmental agencies in the US and in Canada. Other linkages include scientists from the Commission of the European Communities (CEC) that have similar collaborative research programs. Regarding O3, NE-176 scientists (members of academic institutions, USDA-ARS, USDA Forest Service, etc.) bring together a unique diversity of expertise and interests that vary from molecular biology to plant physiology to field studies to numerical modeling. They have a demonstrated record in O3 research and a strong sense of collaboration and scientific integration (see for example, Krupa et al. 2001). Those are the real technical strengths of the project.
Related, Current and Previous Work
Surface level ozone (O3) is everywhere and is the most important phytotoxic air pollutant at the global scale (Krupa et al. 2001). Plant responses to O3 exposures can be acute or chronic. Acute responses are observed in part as visible foliar injury and in part as reversible inhibition of physiological function, when the rates of O3 uptake (from a few to several hours or days) rapidly exceed the capacity for detoxification by the plant (Krupa et al. 2001). Unless acute effects occur as a recurring time series, they may or may not result in adverse effects on growth and productivity (US EPA 1996). Our current knowledge of acute responses of plants to O3 is primarily based on various types of controlled or semi-controlled environmental studies with artificial O3 exposures or field surveys, including the use of sensitive biological indicator plants (Skelly et al. 1997; Krupa et al. 1998; Chappelka et al. 1999). In contrast, chronic responses are products of high and low rates of O3 uptake by the plant throughout the growth season or life cycle, as a random time series involving combinations of stress, avoidance and compensation or repair (Krupa and Kickert 1997). Here, visible foliar injury symptoms may or may not occur. However, reductions in growth and productivity are of primary concern. In contrast to the studies on oxidant-induced classic decline of the forest ecosystem in the San Bernardino Mountains of southern California (Miller and McBride 1998), most exposure experiments have focused on tree seedlings in open-top chambers. In addition to the question of the use of artificial exposure chambers where the micro-climate and constant wind flow patterns (O3 uptake), particularly the latter, clearly differ from the ambient conditions (Jetten 1992), it is difficult to extrapolate the results of the responses of seedlings to mature trees (Samuelson and Kelly 2001) and consequently one should expect differences in stress responses. Nevertheless, research efforts are currently in progress to relate the results between seedlings and mature trees (Kolb et al. 1997) and forests (Laurence et al. 2001). Such efforts need to be significantly expanded. In avoiding the use of open-top chambers, the effects of ambient O3 exposures on the growth of loblolly pine (Pinus taeda) were examined under chamberless conditions, using an O3 protective chemical, ethylene diurea (EDU). Application of EDU increased all growth parameters measured, showing the negative impacts of ambient O3 (W.J. Manning, unpublished). In a separate study, tree level (TREGRO) and stand level (ZELIG) computer simulation models were used to predict species composition in fertile soils and in different climatic zones, with increasing O3 concentrations (Laurence et al. 2001). A 10% decline in red oak (Quercus rubra) abundance and as a result, an increase in sugar maple (Acer saccharum) populations was predicted for southerly climates, with a bearing on biological diversity. In this regard, the effects of O3 on the competitive relationships between native plants showed that the rate of blackberry (Rubus cuneifolus): broomsedge (Andropogon virginicus) litter decomposition was reduced with increasing O3 concentrations, with implications in altering nutrient cycling and thus, biological diversity (US EPA 1996; Kim et al. 1998). Decreased yield and quality of O3-exposed bahiagrass (Paspalum notatum) (Muntifering et al. 2000) and sericea lespedeza (Lespedeza cuneata) (Powell et al. 1999) were of sufficient magnitude to have nutritional implications in their utilization by mammalian herbivores, and potentially altering nutrient cycling, biological diversity and productivity of plant communities. Additionally, exposure to elevated ozone for two years in open-top chambers appeared to reduce species diversity and richness in an early successional plant community (Barbo et al. 1998). One aspect of assessing biological diversity is species fitness or reproductive capacity. Experimental O3 exposures reduced growth and flowering of the butterfly bush (Buddleia davidii) (Findley et al. 1997). Similarly, exposures to O3 reduced flowers and fruits in Spreading dogbane. A very important finding here was that foliar injury was not necessarily required to elicit negative effects on sexual reproduction, thus changes in biological diversity (Bergweiler and Manning 1999). Overall, these considerations are a fundamental part of global climate change and its impacts on vegetation. Environmental, biological and cultural (e.g., irrigation) factors that promote stomatal opening increase the risk of O3 injury to plants. Ozone causes negative effects on a number of plant processes including photosynthesis, water use efficiency, rate of senescence, dry matter production, flowering, pollen tube extension and yield. However, the specific cellular sites that undergo damage are not completely known. For example, photosynthetic activity can be reduced, by decreasing stomatal opening and restricting CO2 uptake, by diminishing energy production in the photosystems or by decreasing CO2 assimilation (Krupa et al. 2001). Although O3 is known to cause stomatal closure, that effect appears to be secondary, since electro-physiological and gas exchange studies show that O3 affects stomatal opening only indirectly in response to K+ fluxes and reduced CO2 assimilation (Fiscus et al. 1997; Torsethaugen et al. 1999). The amount of active carboxylating enzyme, RUBISCO (Ribulose biphosphate carboxylase), can be reduced by O3. Chlorophyll fluorescence measurements have shown that O3 can damage various components of the light-harvesting complex in the chloroplasts. Foliar injury in sensitive plants, reduces the number of functional complexes, diminishing the plant's capability to utilize sunlight for photosynthesis. At lower concentrations, or in tolerant plants, O3 can interfere with the production of electrons, limiting the energy available to "fix" CO2 (Guidi et al. 2000). Although O3 can affect these various functions in the photosynthetic pathway, the sensitivities of the different components, the sequence of events occurring and the specific sites of injury are yet to be identified. In addition to its effect on shoots, O3 is known to adversely affect carbon flow to the root and consequently its biology and biomass (Sane et al. 1996). This has significant consequences for water transport to support gas exchange (Grantz et al. 1999). In this context, use of stable carbon and nitrogen isotopes would be very useful in furthering our understanding (Anderson 2001). Analysis of carbon efflux from primary photosynthetic leaves (Grantz and Farrar, 2000) indicates that phloem loading and the rate of translocation is substantially inhibited by acute exposure to O3. In contrast carbon assimilation was inhibited less substantially. The molecular components of the phloem loading process are possible sites of O3 injury, and potential loci for improved plant tolerance of O3. It is generally accepted that O3 does not persist in the intercellular spaces of the leaf (US EPA 1996). Rather, organic radicals and various reactive forms of oxygen are generated through O3 decomposition and interactions with cell components (Pell et al. 1997). These oxidizing compounds damage proteins and membranes leading to impaired physiological function and cell death. Acute O3 injury in sensitive genotypes, usually seen as development of foliar lesions, resembles the hypersensitive response (HR) of plants to pathogen attack. An oxidative burst occurs as the initial reaction to both O3 exposure and pathogen assault and similar signal molecules have been implicated in induction of the HR and O3 injury (Sandermann 1998). In O3-tolerant genotypes, either the oxidative burst is suppressed (Schraudner et al. 1998) or oxidative damage is highly localized (Koch et al. 2000), thereby restricting the extent of foliar lesions. Since the signal pathways that mediate the response of plants to O3 and pathogens appear to have some common features, our understanding of mechanisms that limit O3 injury could benefit from studies on the molecular aspects of plant-pathogen interactions (Sandermann et al. 1998). The plant antioxidant system (Burkey 1999; Mittler and Zilinskas, 2002), which scavenges naturally occurring reactive oxygen compounds, could function as a primary mechanism to alleviate the oxidative burden resulting from O3 exposure. The ascorbate (vitamin C)-glutathione cycle has been the most intensively studied and generally there is a positive correlation of O3 tolerance with levels of antioxidants and antioxidant enzyme activities (Conklin and Last 1995). For example, mutants that are deficient in vitamin C are very sensitive to O3 (Conklin et al. 1996), as are plants that have been genetically manipulated to produce less ascorbate peroxidase, an enzyme that uses vitamin C to detoxify hydrogen peroxide (Orvar and Ellis 1997). Transgenic plants that have been engineered to overproduce ascorbate-glutathione antioxidant enzymes have provided mixed results regarding O3 tolerance (Mullineaux and Creissen 1999). Ozone induced a rapid increase in adenyl sulfate reductase activity suggesting that redox regulation of specific enzymes may provide a mechanism for rapid response to oxidative stress (Bick et al. 2001). In addition to signaling processes and biochemical protective mechanisms, plants may express differential tolerance depending upon the rate of influx of O3 into the leaf interior. During the late 1970s and mid 1980s, two major O3-crop loss assessment programs were established: The US EPA's National Crop Loss Assessment Network (NCLAN) (Heck et al. 1988) and the European Open-Top Chambers Programme (EOTCP) (Jdger et al. 1993; Fuhrer et al. 1997). Statistical models were developed to define cause-effect relationships. In the United States, one of the main definitions of O3 exposures considered for a 24 hour, 3-month, growing season was a summation function (SUM 06 or sum of all concentrations >60 ppb, US EPA 1996). It was concluded that a 3-month, growing season SUM 06 value of 26.4 ppm7h would protect 50% of the crops in the US NCLAN study from yield losses >10%. In a different comparison, it was predicted that a SUM 06 value of 39.7 ppm7h would protect 75% of the crops in the US NCLAN study from 10% yield loss. Similarly, a 3-month, growing season SUM 06 value of 31.3 ppm7h was associated with <10% biomass reduction in 50% of the deciduous tree seedlings studied. In comparison, a SUM 06 of 42.6 ppm7h was derived for conifer species (US EPA 1996). In Europe, the definition of O3 exposure has been through the use of AOT 40 (Accumulated exposure Over a Threshold of 40 ppb). While SUM 06 represents a sum of all concentrations equal to or above 60 ppb (e.g. 60 + 61 + 62 .....n), AOT 40 is computed as a sum of the difference between 40 ppb and concentrations >40 ppb (e.g. 1 + 2 ...n). Both SUM 06 and AOT 40 are different types of summation techniques of dynamic hourly O3 concentrations that cannot account for the micrometeorological conditions conducive for O3 deposition and uptake and the feedback among plant stress, repair and compensation (Gr|nhage and Haenel 1997; Krupa and Kickert 1997). In the future, emphasis should be placed on the quantification of the 'absorbed dose' which will result in the plant response (Gr|nhage et al. 1997). Furthermore, Bvhm et al. (1995) concluded by using Bray-Curtis ordination analysis that univariate statistics such as SUM 06 were unable to capture up to 39% of the variance in the ambient daily O3 data matrix from 17 sites in the western United States. According to Jordan et al. (1988), the lack of correlation between vegetation exposure statistics, which have been used in modeling ambient air measurements in the United States, has posed a major problem for those trying to assess the effects of ambient O3 exposures. After substantial technical review, the US EPA concluded that the use of a SUM 06 value as a regulatory air quality standard was not advisable. Thus, the current primary (protect human health) and secondary (protect human welfare) National Ambient Air Quality Standards (NAAQS) for O3 in the United States are the same (the annual fourth highest daily maximum 8 h average should be <0.08 ppm) (Federal Register, US, July 18, 1997). This decision has fueled a further need to define and capture the spatial and temporal dynamics of O3 in establishing its mechanistically meaningful relationship to adverse plant responses. Another aspect that deserves much attention, relates to the gaps in our knowledge of the effects of O3 and other associated photochemical oxidants on ecosystems. The US EPA's San Bernardino forest ecosystem study (US EPA 1996) represents one of the best attempts to address the issue and the information gathered there continues to serve as a major source of our understanding. The San Bernardino project was prematurely terminated and full benefits were never realized. However, a number of publications are available on selected aspects of the study (Miller and McBride 1998). These and other ecological studies show that early changes in energy and hydrologic flows due to stress can serve as indicators of ecosystem modification. Here, a small change in one ecosystem component may have amplified effects on other components and the entire ecosystem. An issue unrelated to crop and tree biomass responses, is the identification and use of the typical foliar injury responses of native plant species to ambient O3 exposures as biological indicators to define the spatial and temporal extent of the adverse effects of O3 in national parks and Class I Wilderness Areas (Chappelka et al. 1997; Kohut et al. 1997). This approach is important in natural resource management in the context of environmental impacts. Such studies also have implications in our understanding of the plant community structure and biological diversity (Findley et al. 1997; Bergweiler and Manning 1999). Equally important, biological indicators can be used effectively in developing countries to map the geographic distributions of the occurrence of phytotoxic levels of O3 and other air pollutants, without significant economic and labor costs associated with the use of complex pollutant monitoring equipment. A critical gap in any statistical modeling of the cause and effect relates to the inability of effects scientists to derive an O3 exposure statistic(s) that has an underlying mechanistic meaning (variability in exposure and uptake). In addition to the micrometeorological considerations, different stages of physiological phenology of a given plant species will respond differently to similar or dissimilar O3 concentrations (Krupa and Kickert 1997). A consideration of these aspects has been somewhat controversial, relative to the O3 regulatory aspects and research. Since regulators require simplistic, administratively acceptable explanations of complex processes, until now scientists have been forced to compromise their efforts to improve our process related understanding of the subject matter. In contrast, process models explain the stochastic relationships between the cause and effect purely on a mechanistic basis. In the context of vegetation, results of such studies are spatially limited and scaling the results from the plot level to a region have large associated uncertainties (Kickert and Krupa 1991). Another aspect of process models relates to the use of such models to assess plant responses to air pollution, although initially many of these were developed for some other purposes. Frequently, required validation of the model for its new or different use is a serious limitation. Our current knowledge of the interactive effects of O3 with other plant growth regulating factors is fraught with major uncertainties such as: (a) at best, most experiments are bivariate in nature, a few trivariate, and limited by experimental designs that cannot define the plant response surface fully to satisfactorily explain the nature of the interactions; (b) use inadequate measurements and definitions of the exposure dosimetry; (c) lack adequate measurements of all growth regulating variables; and (d) use inadequate cause-effects models which cannot fully capture the overall mechanistic interactions (Groth and Krupa 2000).
Objectives
-
Describe the spatial - temporal variability of the adverse effects of O<sub>3</sub> on crops and forests.
-
Assess the effects of O<sub>3</sub> on structure, function and diversity of plant communities.
-
Examine the joint effects of O<sub>3</sub> with other growth regulating factors on crop and tree growth and productivity.
-
Examine the molecular and physiological basis of O<sub>3</sub> toxicity and tolerance in plants.
-
Develop numerical models to establish relationships between ambient O<sub>3</sub> exposures and plant responses.
-
Methods
Objective #1 will be addressed through the use of data from continuous O3 monitors and passive samplers and a combination of the use of: (a) foliar injury surveys (e.g., Skelly et al. 1997), (b) biological indicator plants (e.g., Chappelka et al. 1999), (c) open-top chambers (Heagle et al. 1973), (d) protective chemicals such as EDU (Manning 2000); and (e) O3 sensitive and tolerant clones (white clover, black cherry) (Heagle et al. 1994; Lee et al. 1999) or cultivars (bean) (see Manning and Krupa 1992). Scientists from PA and AL have collaborated in field surveys of eastern forests and the use of indicator plants (black cherry, common milkweed and other native plant species) (Chappelka et al. 1999). A similar effort includes AL and NC in the Great Smoky Mountains. Similarly members from NC, VA, MA, OR and NY have collaborated in the use of clover clones (Heagle et al. 1995). Virginia, MA and MN have collaborated in a modeling study on clover (Chevone et al. 1998). For the past year discussions and plant material propagation have been in progress to initiate a multi-membership study (e.g., NC, MA, NY, VA, MD and CA) to utilize O3 sensitive and tolerant bean cultivars (selection of bean cultivars by R.J. Reinert, formerly of USDA) under field conditions to assess the effects of ambient exposures on crop growth and yield. This study will utilize common experimental protocols and crop management practices. Minnesota will perform the cause-effect (multi-point growth series and yield) modeling (see objective #5). In contrast to crops, tree growth responses to O3 will be assessed through both visual foliar injury (PA, AL, NC, CA) and tree ring analyses at sites along the so-called O3 gradients in each study region (scientists at AL, NC, CA, NY-BTI) (also see objective #5). Objective #2 will be addressed at three levels: (a) artificial exposure studies in the greenhouse (Bergweiler and Manning 1999) and in open-top (Barbo et al. 1998) exposure chambers to characterize the O3 response of indicator plant species singly or in mixed cultures (MA, AL); (b) field studies using bio-indicator gardens and the VEGPOP model, for example, in the Great Smoky Mountains (AL, NC); and (c) along a previously identified so-called O3 gradient (NY-BTI, CA). Objective #3 will be addressed using two different approaches for crops: (a) open-top chambers (MD, NC, e.g., Rudorff et al. 1996; Fiscus et al. 1997); and (b) open field ambient exposures (MN, e.g., Krupa and Nosal 1989). The former method is generally used to conduct bi-variate or tri-variate studies, keeping all other independent variables relatively constant. In contrast, in the second approach, locations with differing pollution and plant growth meteorology regimes but with a common plant subject are used, with co-located measurements of many independent variables (see objective #5). Experiments are also underway to determine the effects of O3 and elevated CO2 on decomposition of post-harvest residues of soybean and peanut (NC). For trees, a singletree physiology model (TREGRO) and a stand model (ZELIG) (Laurence et al. 2001) will be used to assess O3 effects and predict forest growth and species composition as a function of available resources (light, moisture, nutrients) (NY-BTI). USDA Forest Service participants from CA have their own in-house model to address these issues. Objective #4 will be addressed at two levels: (a) physiology, and (b) molecular biology. Examples of (a) include: (i) symptom expression, gas exchange and chlorophyll fluorescence in black cherry and green ash (VA), Pima cotton, muskmelon and snap bean (CA), and soybean (NC); (ii) diurnal stomatal conductance curves for key southwestern forest species (CA) and soybean (NC); (iii) analysis of carbohydrate translocation, root development and root respiration on cotton, melon and bean (CA); (iv) tolerant versus sensitive cultivar red-ox states of ascorbic acid in soybean (MD), and apoplastic ascorbate in soybean (MD, NC) and snap bean (NC) (Burkey 1999); and (v) stomatal characteristics of O3 sensitive and tolerant snap bean lines that may contribute to avoidance mechanisms (NC). Molecular mechanisms (#4, b) of O3 sensitivity or tolerance will be addressed through the studies anti-oxidant systems (Mittler and Zilinskas 2002). They include characterization, identification of genetic regulation and construct of transgenic plants to test for the molecular basis of tolerance versus sensitivity to O3 (Bick et al. 2001) (NJ, VA). Here a number of plant species will be used, they include Arabidopsis, bean, tobacco, tomato and spinach. Objective #5 for crops will use a growth model combined with a multi-variant, non-parametric model of yield, initially for alfalfa (modified from Krupa and Nosal 1989). In an ambient field plot study at multiple sites, data on O3, SO2, NOx, meteorological variables and alfalfa growth and yield have already been gathered for 48 harvests and another 20 harvests are planned. This model will be modified for use in the multiple-participant bean study (objective #1) (MN). For trees, the TREGRO singletree model incorporates experimentally determined effects on leaf photosynthesis to simulate effects on leaf area, tree height and root growth. Ozone responses of 2-5 species will be evaluated in TREGRO for each forest stand (total 35) modeled in ZELIG using monitored hourly O3 and meteorological data (BTI-NY). In addition, in CA, Forest Service scientists will use their in-house statistical model.Measurement of Progress and Results
Outputs
- Over the course of the project, spatial and temporal patterns of crop and tree responses (foliar injury and/or changes in growth and productivity) to O<sub>3</sub> will be described and mapped across the northeast region and other areas to assess the extent of regional scale adverse impacts.
- Over the course of the project select indicator native plant species fitness (indicative of biodiversity) and changes in community structure due to O<sub>3</sub> exposures will be described. Impact of O<sub>3</sub> on biodiversity will be quantified and information transferred to natural resource managers.
- Identified crop and tree responses to the interactive effects of O<sub>3</sub> with other growth regulating factors will be used to predict production changes over time, including changes relevant to climate change, with the goal of transferring such information to both public and private urban and industry planners.
- Information on the mechanisms of O<sub>3</sub> toxicity and tolerance in plants and alterations in their functional physiology to O<sub>3</sub> exposures will lead to the identification of genes that can mitigate the effects of O<sub>3</sub> and other forms of oxidative stress, assisting in developing and/or in identifying tolerant cultivars and varieties for the production sector.
- Over the course of the project new statistical methodologies and derived information will establish relationships between O<sub>3</sub> exposures and plant responses that explain the randomness (spatial and temporal variability) of such relationships. Ultimately such information will be transferred to regulatory policy makers.
- Output 6. Data derived from the project will be used to establish limits of O<sub>3</sub> that have an immediate bearing on the National Ambient Air Quality Standards for O<sub>3</sub>.
Outcomes or Projected Impacts
- Scientific basis for developing National Ambient Air Quality Standards for O<sub>3</sub> to protect cultivated (crops) or managed (forests) and native vegetation (bio-diversity).
- Environmental and quality of life benefits through post-regulatory (air quality standards) impact assessment.
- Improved understanding of regional scale issues in resource management.
- Improved understanding of plant response to oxidative stress (drought, herbicides, pathogens, etc.), assistance in developing tolerant plants through breeding and other approaches.
- Early detection of ecosystems under O<sub>3</sub> stress.