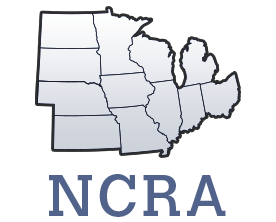
NC_old1203: Lipids In Plants: Improving and Developing Sustainability of Crops ("LIPIDS of Crops")
(Multistate Research Project)
Status: Inactive/Terminating
NC_old1203: Lipids In Plants: Improving and Developing Sustainability of Crops ("LIPIDS of Crops")
Duration: 10/01/2016 to 09/30/2021
Administrative Advisor(s):
NIFA Reps:
Non-Technical Summary
Statement of Issues and Justification
The need The North Central (NC) committee, Lipids In Plants: Improving and Developing Sustainability of Crops (or “LIPIDS of Crops”) will work together to characterize lipid-related metabolism and traits relevant for crop improvement and to develop crops with improved yield, biotic and abiotic stress tolerance, and/or nutritional and industrial qualities. Lipids play critical structural, metabolic, and regulatory roles in all aspects of plant growth and development and in responses to environmental challenges. In addition, plant oils are an important source of nutrition for humans and livestock, and their trade generates billions of dollars annually. Dependence on plant oils for renewable chemical feedstocks and biofuel uses has also increased. Despite the importance of plant lipids, many fundamental questions remain unanswered about lipid compositions, metabolism, and function, impeding improvement of crop productivity and oilseed yields and fatty acid quality for human and livestock nutrition and industrial uses. The importance of the work Each plant is a green factory that uses solar energy and carbon dioxide to produce tens of thousands of compounds. Many thousands are non-water soluble compounds called lipids; these include photosynthetic pigments that harvest sunlight, building blocks of cellular membranes, protective and structural materials, seed oils, vitamins, plant (phyto-) hormones, signaling molecules, and essential oils that attract pollinators or repel insect and microbial predators. Despite their biological and economic importance, lipids are the least explored central metabolites. Limited understanding of plant lipid metabolism and function limits our ability to increase production of food and renewable resources for the growing population. In fact, the physiological functions of many plant genes putatively encoding lipid-metabolizing enzymes are unknown and their substrates are not defined. Although many plant lipids function as hormones and signaling messengers, the regulatory roles and networks through which lipids mediate plant growth and development are woefully unclear. Answers to these questions are essential for informed crop improvement through breeding and biotechnology. The technical feasibility of the research LIPIDS of Crops will improve and extend methods for lipid characterization and measurement, identify and characterize lipid-related metabolism and traits relevant for crop improvement, and develop crops with improved yield and/or functionality. Approaches for lipid analysis will include mass spectrometry, imaging approaches, and flux analysis. One hindrance to significant expansion of current knowledge of plant lipids is less-than-adequate methodology to address central questions about lipid metabolism and lipid function. LIPIDS of Crops members will combine forces and share information to improve and implement lipid analytical strategies that will advance understanding of plant metabolism, regulation, development, and stress response, and enable plant improvements to increase food, feed, and seed oil production. Approaches for identifying and characterizing lipid-related traits will include analysis of genetic variants in lipid-related genes, biochemical approaches, and physiological analyses. Development of crops with improved yield and/or functionality will be via marker-assisted breeding or transformation with the relevant genes. Initial group members have the relevant technical capabilities and access to instrumentation to pursue all aspects of the research. The advantages of doing the work as a multistate effort The multistate effort will provide a framework to enhance collaboration and sharing of resources, expertise, and instrumentation to accelerate progress among the high concentration of plant lipid researchers in the North Central Region. Likely impacts of the work The impacts will be • a collaborative plant lipid research group, poised to participate in new opportunities • more efficient analytical pipeline to obtain information on lipid metabolism and traits relevant for crop improvement, • better understanding and improvement of plant growth under biotic and abiotic stress conditions • knowledge of seed metabolism and biology for improvement of seed oils • improvement of crop productivity and quality for human and livestock nutrition and the bio-based economy.
Related, Current and Previous Work
Information in CRIS and in the literature has been reviewed and incorporated into this section. While individual and small group projects described in CRIS deal with lipid analysis, lipid metabolism, and seed oil improvement, there is no project that incorporates all these themes. The multistate group will work together to improve tools and understand lipid metabolism, and to bring the tools and knowledge to bear on crop improvement. 1. Methods for lipid characterization and measurement Lipidomic analyses The term “lipidomics” describes comprehensive analysis of the lipids in a tissue or other sample, typically by mass spectrometry. “Lipidome” refers to the entire array of lipid molecular species present in a cell, tissue, organism, or other biological unit. In the past fifteen years, mass spectrometry-based lipidomics has given rise to extensive data on the range of lipids present in the model plant Arabidopsis thaliana, including glycerolipids, sphingolipids, sterol derivatives, storage lipids, cuticular lipids, and others (Markham et al., 2007; Perera et al., 2010; Ibrahim et al., 2011; Samarakoon et al., 2012; Schrick et al., 2012; Okazaki et al., 2013; Koo et al., 2014; Li et al., 2014a; Nilsson et al., 2014). Many of these data were obtained by members of this multistate group. Among group members, the Cahoon, Durrett, Koo, Lee, Markham, Miernyk, Nikolau, T. Wang, X. Wang, and Welti use mass spectrometry directly to obtain lipid compositional data, while nearly all groups utilize such data. Individual groups use specific technologies (types of mass spectrometers) and approaches (e.g., direct infusion or liquid chromatography) and have unique abilities to characterize specific lipids. For example, Nikolau’s group specializes in characterization of lipids found in plant cuticles while Markham’s group specializes in sphingolipids. While considerable information on Arabidopsis is available, at this time, comprehensive data on crop plant lipids are just beginning to be assembled (Riedelsheimer et al., 2013; Narayanan et al., 2015a; 2015b) and data on most crops, as well comprehensive data, are lacking. Similarly, while analytical methods have been developed for many abundant classes of lipids, analyses of many lipid intermediates and other minor lipid species have not been developed. Additionally, available analyses have not been integrated to provide an all-inclusive view of lipid metabolism. Visualizing and localizing lipids Approaches to localizing specific lipids in plant cells and tissues include mass spectrometry-based imaging and visualization of fluorescently labeled lipids. Mass spectrometry imaging technology can be used to decipher lipid localization at high-spatial resolution directly on plant tissues (typically off the plant). The Lee group is a key developer of this technology, which can now map lipids at ~10 µm resolution (Horn et al., 2012; Lee et al., 2012). Nikolau’s and Wurtele’s groups also use MALDI-MS to evaluate the spatial distribution of lipids (Cha et al., 2008; Korte et al., 2015). On the other hand, fluorescent labeling has the potential to visualize lipids in situ. Although many dyes and/or probes are available for broad classes of biomolecules, the ability to directly label and view specific lipids is just beginning to emerge. Schrick’s group has adapted a protocol, originally developed for mammalian cells (Jao et al., 2009; Jao et al., 2015a; 2015b), for fluorescence imaging of choline phospholipids in plants by labeling with propargylcholine, a choline analog, coupled with click chemistry using fluorescein azide (Paper and Schrick, unpublished). Data on plant lipids The vast majority of lipid characterization data are not deposited in a database currently. Plant/Eukaryotic and Microbial Systems Resource (PMR: http://metnetdb.org/PMR/) is a database designed for archiving, analyzing, and disseminating metabolomic and transcriptomic data and associated metadata by the Wurtele lab (Hur et al., 2012). PMR provides for analysis and comparison within and across experiments, and transcriptomics data can be co-analyzed with metabolomics data. Currently PMR contains data from Nikolau, Welti, and Wurtele labs, as well as from over 20 other US and international research groups. 2. Lipid-related metabolism and traits relevant for crop improvement Roles of lipids and lipid-related gene products Roles of lipids in plants include forming membrane bilayers and plant cuticles, storing energy, particularly in seeds, and serving as signals involved in plant development, growth, and stress responses. Despite the importance of lipids in plants, large gaps remain in our knowledge of plant lipid metabolism. In the following paragraph, the state of knowledge for lipid metabolism in the model plant Arabidopsis thaliana will be described. Though there are many gaps in our knowledge of Arabidopsis lipid metabolism and the gene products that catalyze it, even less specific information about lipid metabolism is available for crop plants. In Arabidopsis, over 900 genes have been identified as putatively involved in lipid metabolism (aralip.plantbiology.msu.edu; Li-Beisson et al., 2013, Troncoso-Ponce et al., 2013). Although we know that lipid metabolism is very dynamic with compositions changing diurnally, rapidly in response to stress, and during development (Kilaru et al., 2012; Nakamura et al., 2014; Vu et al., 2014), many enzymes acting at specific developmental time points are not defined, nor, in most cases, are the relative fluxes through co-existing enzymes and pathways. In fact, less than 300 Arabidopsis lipid-metabolizing gene products have been characterized biochemically or by mutant analysis, and knowledge of the rest depends primarily on DNA sequence similarities to characterized genes from other species. Beyond this, there are about 30 lipid-related biochemical activities with no proposed relationships to genes (aralip.plantbiology.msu.edu; Li-Beisson et al., 2013), suggesting that there are additional lipid-metabolizing genes not yet identified. Additionally, there are many examples of lipid-metabolizing genes that encode proteins with similar sequences, but different functionality, indicating that detailed genetic, biochemical, and physiological analysis will be required to understand functions of lipid related genes. For example, 300 genes encode lipases (i.e., lipid-hydrolyzing enzymes) (Troncoso-Ponce et al., 2013). Current knowledge suggests that these enzymes vary in terms of the range of substrates that they hydrolyze in planta and also in the timing and location of their expression. For example, X. Wang’s group has characterized 12 phospholipase Ds (PLDs) that hydrolyze the alcohols from phospholipid head groups. Individual PLD family members play distinctive roles in germination and seed viability, seed oil composition, stress tolerance, root hair patterning, pollen tube growth, and senescence (Li et al., 2009). One particularly well-characterized PLD, phospholipase D?1, acts in an abscisic acid-initiated signaling cascade via a protein phosphatase to trigger stomatal closure (Li et al., 2009). To add to the complexity, interactions among various lipid metabolizing enzymes can regulate functionality. For instance, Wang and Markham demonstrated interplay between sphingolipid-metabolizing and phospholipid-metabolizing enzymes in regulation of stomatal closure in response to abscisic acid (Guo et al., 2012). Thus, lipid-metabolizing enzymes perform functions critical to proper plant growth and development, seed oil formation and its regulation, and tolerance to biotic and abiotic stressors, but the much remains to be understood even in the best-studied model plant species. Clearly, knowledge also needs to be extended to crop plants, so that lipid-related information can be used to optimize crop performance, yield, and functionality. Characterization of mechanisms and functions of lipid-related traits Virtually all members of the “LIPIDS of Crops” group are involved in characterization of lipid-related traits in plants. The X. Wang and Welti groups characterized the function of a number of lipases that are involved in stress and seed metabolism (Zien et al., 2001; Welti et al., 2002; Li et al., 2004; Li et al. 2006; 2011; 2013; Hong et al., 2008; 2009; Peters et al., 2010; Yang et al., 2012). Roston has characterized a galactolipid-remodeling enzyme that mitigates freezing damage and a lipid transporter (Roston et al. 2011; 2012; 2014; Vu et al., 2015). The Cahoon, Markham, and Stone groups have characterized genes involved in sphingolipid metabolism; these regulate cell growth, stomatal opening, and the induction of cell death in the plant immune response (Kimberlin et al., 2013). Schrick’s lab is analyzing putatively lipid-binding transcription factors that regulate cell-type differentiation in plants (Schrick et al., 2004; Schrick et al., 2014) and, along with Welti, has investigated the role of enzymes involved in production of sterol derivatives that vary in level during plant stress responses (Schrick et al., 2012; Narayanan et al., 2015). Louis, Koo, Hoffmann-Benning, and their colleagues have identified and characterized proteins involved in lipid signaling in response to abiotic and biotic stressors, including insects and microbial pathogens (Louis et al., 2010; Guellette et al., 2012; Nakata et al., 2013; Koo et al., 2014; Smith et al., 2014; Zhang et al., 2015). Allen, Thelen, Miernyk, Durrett, Cahoon, Nikolau, X. Wang, and Wurtele are working to understand steps in lipid synthesis that are critical to filling of oil seeds (e.g., Durrett et al., 2010; Hajduch et al., 2010; Lu et al., 2011; Ngaki et al., 2012; Manandhar-Shrestha et al., 2013; Guo et al., 2014; Liu et al., 2015; Li et al., 2015a). Additionally, Cahoon and Durrett are characterizing activities that encode enzymes catalyzing the formation of unusual, chemically active, and valuable fatty acids for potential incorporation into crop plants (e.g., Durrett et al., 2010; Kim et al., 2015a; 2015b; Malik et al., 2015; Nguyen et al., 2015). Whereas many genes that function in central lipid metabolism have been known for some time, perhaps surprisingly, others have been discovered only recently, and the roles of many genes in major lipid synthetic pathways still are not fully understood (e.g., Lu et al., 2009; Zhang et al., 2009; Ngaki et al., 2012; Fan et al., 2014). On the other hand, genes involved in lipid signaling have, in comparison, only begun to be revealed and it is likely that many of the large number of unstudied, putatively lipid-related, genes may play signaling or membrane remodeling roles. Approaches to identify genes with potentially lipid-related activities include proteomic analysis to identify proteins present at the site of lipid-associated activities, a strategy used by the Thelen, Miernyk, and Hoffmann-Benning groups (e.g., Bräutigam et al., 2008; Hajduch et al., 2010; 2011). Via this approach Miernyk and coworkers identified SEIPIN proteins in soybean oil bodies during the latter stages of seed maturation/desiccation. Recently it was shown that ectopic overexpression of SEIPIN1 in Arabidopsis resulted in increased numbers of large oil bodies in leaves, as well as in seeds, and increased seed oil content by up to 10% over wild-type seeds (Cai et al., 2015), making this a promising candidate for increasing oil content. Another approach to identifying lipid-related genes is analysis of lipid-related traits in relation to genetic variation. An example is a genome wide association study for lipid-related traits (Riedelsheimer et al., 2013). Analysis of insertional and chemically induced knockout and knockdown mutations and ectopic or overexpression of genes putatively associated with lipid metabolism are frequently used strategies. Mutations or changes in expression can be analyzed in terms of effects on plant physiology, development, lipid composition, and/or lipid localization. These approaches are used routinely by Cahoon, Durrett, Hoffmann-Benning, Koo, Louis, Markham, Nikolau, Roston, Schrick, Stone, Thelen, X. Wang, and Wurtele groups (e.g., Li et al., 2004; Li et al., 2011; Koo et al., 2014; Li et al., 2015b; Zhang et al., 2015). These methods may be complemented with expression of a protein of interest in bacteria or yeast to analyze enzyme activity in vitro, as demonstrated by work in the Cahoon, Koo, Schrick, and Wang groups (e.g., Yang et al., 2011; 2012; Koo et all, 2014; Stucky et al., 2015; Zhang et al., 2015). A tool that has been very useful in discovering new pathways in central lipid metabolism, which can be applied to lipid metabolism in higher plants, is flux analysis, which is performed by the Allen group (Ma et al., 2014; Allen et al., 2015). Flux analysis in combination with mutant analysis can aid in defining the role of specific gene products in lipid-metabolizing pathways (e.g., Xu et al., 2003). 3. Crops with improved yield and/or functionality Traits that increase quantity of plant products Vegetable oils, consisting primarily of triacylglycerols (TAGs), are major caloric sources in human and livestock diets and combustible fuel sources for biodiesel and jet fuel production. Vegetable oils are also widely used as a medium for fried food preparation, and the fatty acid components of vegetable oils can serve as sustainable hydrocarbon feedstocks for industrial applications, including lubricants and nylon precursors that are currently obtained from petrochemicals (Lu et al., 2011). An increasing global demand for vegetable oils to supply these expanding markets has resulted in a critical need to increase vegetable oil production per acre and to develop new vegetable oil sources to supplement those currently obtained largely from seeds and fruit mesocarps (Lu et al., 2011). Research in several labs of this multi-state group has been directed at meeting the global vegetable oil demand. The X. Wang and Clemente labs, for example, have explored approaches to enhance carbon and fatty acid flux into the synthesis of TAGs by seed-specific expression of key metabolic genes and transcription factors such as Wrinkled1 (Wri1) in camelina and soybean (Clemente and Cahoon, 2009; Li et al., 2013; Lu et al., 2015). The Thelen lab has examined the regulation of acetyl-CoA carboxylase, a rate-limiting enzyme that provides the malonyl-CoA building block for fatty acid synthesis (Thelen and Ohlrogge, 2002; Chen et al., 2009). Furthermore, the Clemente lab has conducted research to express genes for TAG biosynthesis and storage in sorghum leaves and stalks to generate vegetable oils as co-products of lignocellulosic biomass. Moreover, the Allen group’s protocols for carbon flux analysis and measurement of biosynthetic intermediates are expected to increase the predictability of biotechnological and breeding approaches for enhancement of TAG production in seeds and other plant organs (Allen et al., 2014; Allen et al., 2015). Crops with increased quality of plant products Modification of fatty acid compositions and fatty acid storage forms offer opportunities for enhancing the functionality and value of vegetable oils in sorghum, camelina, and soybean. The Cahoon and Clemente labs, for example, have engineered soybeans to produce long-chain omega-3 polyunsaturated fatty acids (PUFAs) and carotenoids for use of soybean as a sustainable, land-based source of aquaculture feed (Clemente and Cahoon, 2009). The Clemente lab has also modified levels of fatty acid unsaturation in soybean seeds to produce a vegetable oil formulation for soft-spread margarine without the need for hydrogenation (Graef et al., 2009; Park et al., 2014). Similarly, the Cahoon lab has conducted extensive metabolic engineering in camelina to generate vegetable oils with novel fatty acid compositions for use as sustainable substitutes to petroleum as fuel and industrial feedstocks (Kim et al., 2015a; 2015b; Nguyen et al., 2015). To complement biotechnological approaches, the Cahoon and Durrett labs are actively involved in screening mutant camelina germplasm for altered fatty acid profiles to identify breeding lines that can be used to produce oils with improved oxidative stability for food processing and biodiesel applications. In addition to research aimed to enhancement of fatty acid compositions, the Cahoon and Durrett labs have developed camelina lines that accumulate alternative seed oil storage forms, including wax esters for lubricants and acetyl-TAGs for drop-in biodiesel (Durrett et al., 2010; Liu et al., 2015). Similar approaches are being used to tailor membrane structures of plant cells for improved tolerance to biotic and abiotic stresses. The plasma or outer membrane of plant cells provides the primary barrier to bacterial, fungal, and insect pathogens and to osmotic adjustments associated with drought and freezing. In addition, membranes provide a repository of lipids that can generate signal molecules for localized or distal responses to environmental perturbations. Recognizing membranes as key targets for crop improvement, the Markham and Clemente labs have modified sphingolipid composition in sorghum and soybean to enhance cold tolerance and potentially broaden the temporal and geographic ranges for production of these crops. Rational modifications of sphingolipid metabolism are also being pursued by the Markham and Cahoon labs to improve resistance to bacterial and fungal pathogens. In addition, the X. Wang and Louis labs have also developed strategies for altering production of signaling lipids, such as phosphatidic acid (PA), for improved drought and insect tolerance (Li et al., 2009; Peters et al., 2010; Yang et al., 2012; Lu et al., 2013; Zhao et al., 2013).
Objectives
-
Improve and extend methods for lipid characterization and measurement.
-
Identify and characterize lipid-related metabolism and traits relevant for crop improvement.
-
Develop crops with improved yield and/or functionality.
Methods
Objective 1: Improve and extend methods for lipid characterization and measurement (Hoffmann-Benning, Lee, Markham, Schrick, T. Wang, X. Wang, Welti, Wurtele; IA AES, KS AES, MI AES, NE AES, Donald Danforth Plant Science Center, Iowa State University). Extend and improve lipidomic analyses We will increase knowledge of the lipidomes of crop systems, increase capabilities for quantifying crop lipids, and increase analytical throughput to support projects that require analysis of large numbers of samples. The multistate group will use mass spectrometry to identify lipid species present in crop plants, including soy, sorghum, and camelina. This work will be performed primarily by the Markham, X. Wang, and Welti groups. Initially, we will take will take a survey approach using mass spectral scanning of intact lipid ions. We will catalog lipid fragment ions observed in a survey of product ion scans at varied collision energies. Identified intact ion masses will be annotated and unknown lipid ions will be investigated by further product ion analysis. The Welti, X. Wang, Miernyk, and Markham groups will share plant materials and combine results to define comprehensive lipidomes for soy, sorghum, and camelina leaves and seeds. Quantitative analyses will be formulated for the identified lipid molecular species of crop plants, using direct infusion and/or lipid chromatography approaches. In general, direct infusion (e.g., Welti et al. 2002) is simple and appropriate for analysis of lipids present in relatively large amounts, whereas liquid chromatography (e.g., Markham et al., 2007; Guelette et al., 2012) can add specificity and sensitivity for less abundant lipids. A major barrier to quantification of lipids is the availability of appropriate internal standards. Members of “LIPIDS of Crops” will share internal standard mixtures, quantification protocols, and other know-how. To associate lipid metabolic functions with crop traits and their genetic underpinnings, analysis of large numbers of genetically variant plants may be required. Lipid analysis of large numbers of crop samples would be more feasible if current costs were decreased. To decrease costs, given the high costs of personnel and mass spectrometers, methods need to be more rapid. One area targeted for improvement is the extraction of lipids from plant material. Generally rapid extraction methods involve one or a few extraction with a solvent mixture more polar than used in traditional extraction methods (e.g., Vu et al., 2014). Such approaches for crop plant materials will be optimized by the Markham and Welti groups, using current extraction approaches as benchmarks, and finally automating to the greatest extent possible. Additionally, development of abbreviated mass spectrometry methods that capture key lipid precursors, intermediates, and products of each metabolic pathway will be developed for large-scale experiments on crop plant materials. Members of “LIPIDS of Crops” will share insights from their work to define key molecular species and potentially reduce analysis of metabolically redundant species (e.g., Vu et al., 2014 for identification of co-metabolized lipids). Welti directs the Kansas Lipidomics Research Center, which can make rapid analyses available to the entire group and other members of the scientific community. Visualize and localize lipids Lipids will be visualized and localized in plant tissues using two approaches, mass spectrometry-based visualization and fluorescent labeling of lipids. Mass spectrometry imaging technology to decipher lipid localization at high-spatial resolution directly on plant tissues will continue to be developed in the Lee lab. Modifications will be made to the mass spectrometer set-up to enhance resolution and sensitivity to the level of single cells. The technology will be applied to lipids in crops via collaborations with team members (Objective 2) to better understand the intra-tissue organization of lipid biosynthesis. Extension of fluorescent labeling protocols to cover membrane constituents such as sterols and steryl glucosides will be developed for plants in the Schrick lab. These imaging tools, which will inform subcellular localization of specific lipids in plants, are complementary to high spatial resolution MALDI imaging. Develop a system for archiving, analyzing, and disseminating data The “LIPIDS of Crops” multistate group plans to utilize the Plant/Eukaryotic and Microbial Systems Resource (PMR; http://metnetdb.org/PMR/) for archiving, analyzing, and disseminating data resulting from group activities. The PMR database has excellent functionality for integration with other “omics” data. Plant/Eukaryotic and Microbial Systems Resource is a project of the Wurtele group, who will develop a completely automated mechanism for upload of data and associated metadata. The Wurtele group will develop application program interfaces to link PMR to other bioinformatics sites. For example, Arabidopsis metabolomics data in the PMR database will also be included in Araport (www.araport.org) and that and other data will be copied in the Metabolomics Workbench database. Objective 2: Identify and characterize lipid-related metabolism and traits relevant for crop improvement (Allen, Cahoon, Durrett, Hoffmann-Benning, Koo, Louis, Markham, Miernyk, Nikolau, Roston, Schrick, Stone, Thelen, X. Wang, Welti, Wurtele; IA AES, KS AES, MI AES, MO AES, NE AES, Donald Danforth Plant Science Center, USDA-ARS/Missouri) In tackling Objective 2, the LIPIDS of Crops group will utilize the lipid analysis, lipid localization, data storage, and data analysis tools generated in Objective 1 as components of their approaches to identify and characterize lipid-related metabolism and traits in plants. Knowledge about the function of specific genes and their products will be utilized for crop and seed oil improvement in the work described in Objective 3. The group will use multiple approaches to identify gene products that affect lipid metabolism and traits relevant to crop growth, stress responses, and oil production. Some researchers will analyze the function of genes that have been tentatively identified as lipid-related. For example, Markham and Cahoon will analyze the function of genes involved in sphingolipid metabolism, in particular, genes related to the synthesis of ceramide and ceramide-1-phosphate, lipids potentially important in the response of plants to pathogens. Louis’ and Koo’s groups will analyze genes producing lipids and/or lipid-derived metabolites that have defensive functions in plant-insect or plant-pathogen interactions (Louis et al., 2010; Koo et al., 2011; 2014; Sanjaya et al., 2013; Marti et al., 2015; Zhang et al., 2015). They will identify crop orthologs of key genes with functions known in Arabidopsis to control innate defense against insects and fungal pathogens. The Hoffmann-Benning group will characterize products of crop genes which encode lipid-interacting proteins changing plant development in response to abiotic stress, particularly drought. They are currently investigating a group of PA-binding proteins including lipases and lipoxygenases, one of which enhances drought tolerance and seed yield. Roston will analyze lipid-metabolizing genes whose functions putatively affect cold and freezing responses in Arabidopsis and in maize and related grasses. Schrick will analyze homeodomain leucine-zipper (HD-Zip) transcription factors with Steriodogenic Acute Regulatory protein (StAR)-related lipid transfer (START) domains, involved in regulation of plant development. Nikolau, Miernyk, and Thelen will study genes encoding enzymes that produce the lipid building blocks, acetyl CoA and malonyl CoA. Nikolau and Cahoon will also study enzymes involved in production of novel lipid molecules with physical and chemical properties pertinent to nutrition or industrial applications. The Miernyk group will express SEIPIN proteins first in Arabidopsis and then in soybean to determine their effects on oil body structure and oil accumulation. Members of the “LIPIDS of Crops” group also will use proteomic methods to detect lipid-related gene products. Thelen will investigate proteins that bind to and phosphorylate acetyl-CoA carboxylase, to better understand the committed step in de novo fatty synthesis. Miernyk will continue to identify proteins that regulate triacylglycerol levels in developing soybeans (Smith-Hammond et al., 2014). Hoffmann-Benning will use proteomic data to identify lipid-binding proteins in plant phloem (Guelette et al., 2012). Allen lab will perform flux analysis of leaves and seeds of crop plants to identify relative flux through particular pathways. From these data Allen will predict the gene products that allow for major fluxes and those that present bottlenecks. It’s also possible that previously unknown metabolic pathways will be discovered. Predictions as to the role of particular enzymes in producing the observed flux will be tested by genetic alteration of those enzymes in cooperation with the Durrett lab. Additionally, Welti, Koo, and X. Wang will perform a genome wide association study in Arabidopsis to identify new genes that affect lipid composition. Welti also will analyze sorghum mutants to identify genes that affect lipid levels (Xin et al. 2008). The tools in PMR also will provide a key resource to identify new genes of lipid metabolism in Arabidopsis and crop species and to develop testable hypotheses about the function of genes identified by any method (e.g., Yeo et al, 2012; Fukushima et al. 2014; Li et al., 2015b; Li and Wurtele, 2015.). To analyze the function of lipid-related genes, known-down (e.g., RNAi) or knock-out mutants and lines exhibiting over-expression will be analyzed for physiological function, biochemical function, and lipid compositional differences as compared to wild-type lines. Proteins will also be assessed by expression in yeast or bacterial systems, as appropriate. In particular, biochemical activities associated with in vitro-expressed proteins will be assessed, and hypotheses about substrates and products will be developed, by using a comprehensive lipidomics approach to measure lipid composition before and after the proteins are incubated with plant lipids. Substrate requirements will then be ascertained more completely by incubating the enzymes with various purified lipids and combinations of purified lipids. Objective 3. Develop crops with improved yield and/or functionality (Allen, Cahoon, Clemente, Durrett, Hoffmann-Benning, Markham, X. Wang; KS AES, MI AES, NE AES, Donald Danforth Plant Science Center, USDA-ARS/Missouri) Incorporate traits that increase quantity of plant products into sorghum, camelina, and soybean The multistate project is anticipated to more rapidly advance biotechnological and breeding efforts to improve the quantity and quality of plant products by bringing together complementary expertise and resources that are not available in any one location. In addition, findings from Objective 2 will provide a basic understanding of plant lipid metabolism to enable more precise and rational strategies for crop improvement in this Objective. Advanced methods for lipidomic analyses developed in Objective 1 will allow a more global assessment of intended and unintended impacts of specific biochemical alterations on lipid metabolic networks to further guide biotechnological and breeding approaches for plant product quantity and quality enhancement. As part of this research, the Allen lab will use existing flux maps of camelina and soybean seeds to predict bottlenecks in central metabolism that constrain oil production. Working in collaboration with the X. Wang and Clemente labs, metabolic engineering strategies will be developed and implemented to overcome these bottlenecks. Engineered camelina and soybean lines will be subjected to oil content measurements and flux analyses in the Allen lab to determine the effectiveness of predictions for addressing metabolic bottlenecks. Based on these findings, iterative improvements in metabolic engineering strategies will be implemented. It is expected that results from these studies will also inform soybean and camelina breeding efforts. Based on numerous studies, it is unlikely that large increases in seed oil content will be obtained by altered expression of single genes. The X. Wang lab will initially test multigenic combinations or gene stacks, based in part on flux analyses studies, using camelina as a host. It is expected that the non-labor intensive genetic transformation protocol for camelina, in combination with its relatively short growing season, will facilitate rapid testing of gene stacks for increased seed oil content. Gene combinations that show the most efficacy in camelina will be introduced into soybean by the Clemente lab. Studies will be conducted in parallel using similar flux map-based approaches to enhance vegetative oil production in sorghum, as a collaboration between the Clemente and Allen labs. It is anticipated that these studies will broaden from current efforts to increase TAG biosynthesis to encompass down-regulation of catabolic pathways that also limit TAG accumulation. Incorporate traits that increase the quality of plant products into sorghum, camelina, and soybean The Cahoon and Durrett labs will collaborate to introduce FatB thioesterases and divergent lysophosphatidic acid acyltransferases (LPATs) into acetyl-TAG-producing camelina lines. The goal of these studies is to generate oils enriched in low viscosity acetyl-TAGs for improved drop-in biodiesel and lubricant functionalities (Kim et al., 2015a; 2015b). In addition, studies in Objective 2 directed at understanding acetyl-CoA biosynthesis will provide the basis for studies to increase acetyl-CoA substrate pools in seeds for increased acetyl-TAG production. Promising lines arising from these studies will be grown in large plots in the Nebraska biotech field facility to obtain sufficient amounts of seed for oil functionality testing. The Cahoon and Durrett labs will also work collaboratively to screen camelina mutant populations for altered seed oil compositions, including those with reduced polyunsaturated fatty acid content. Mutations in selected lines will be mapped and crosses conducted to combine mutant alleles for desired oil compositions. It is expected that these backgrounds will be combined with those previously described to produce elite germplasm with increased total oil content and optimized fatty acid composition. The X. Wang lab will optimize approaches developed in model species using diverse phospholipase genes to fine-tune membrane compositions and production of membrane-derived signaling molecules, including PA (Peters et al., 2010; Li et al., 2014b). It is anticipated that these approaches will contribute to improved seed and biomass production and improved biotic and abiotic stress resistance. Studies will initially focus on camelina, and approaches found to be most effective will be extended to sorghum and soybean in collaboration with the Clemente lab. Studies focused on modulating PA production will be conducted with the Hoffmann-Benning lab to probe phloem transport of PA to distal portions of the plant as a mechanism for improving tolerance to stresses, such as drought (Benning et al., 2012). The Markham and Clemente labs will build on promising preliminary data to refine strategies for expression of sphingolipid fatty acid desaturases in sorghum and soybean to enhance cold tolerance of these crops without compromising agronomic performance. These will be tested under field conditions with staggered spring planting dates, including those earlier than normally used for these crops. The Markham and Cahoon labs will also translate approaches developed in Arabidopsis to alter sphingolipid homeostatic regulation for increased biomass and enhanced heat and bacterial pathogen resistance in camelina, soybean, and sorghum (Kimberlin et al., 2013). Efforts will also be initiated to combine oil traits with agronomic traits that improve productivity of soybean, camelina, and sorghum enhance the value and field performance of these crops. To speed these studies, synthetic biology tools will be implemented that enable precise genome modifications and more rapid assembly and delivery of multiple, diverse trait genes. Methods such as CRISPR/Cas9 or RNAi will be applied for knockout of specific genes that constrain oil production or confer synthesis of non-desired fatty acid and lipid compositions for oil quality and membrane properties (Carmichael et al., 2015). For multi-gene assembly, methods such as GoldenBraid 2.0 (Sarrion-Perdigones et al., 2013; Sarrion-Perdigones et al., 2014) will be used for modular and interchangeable trait gene assembly.Measurement of Progress and Results
Outputs
- Comprehensive and improved rapid methods of lipid analysis by mass spectrometry
- Improved methods of lipid visualization and localization in plant tissues of lipids not previously visualized
- Lipidomic data of entire “LIPIDS of Crops†group available to scientific public via PMR database
- Identification and characterization of previously uncharacterized genes involved in lipid metabolism in model and crop plants, including establishment of linkages between lipid-related genes and specific aspects of oil accumulation and stress response or resistance
- Camelina plants with oil composition optimized for one or more specific applications
- One or more transgenic crop plants with improved tolerance to one or stresses
- Soybeans with increased lipid content, decreased raffinosaccharide content, and unchanged total protein levels
Outcomes or Projected Impacts
- A collaborative plant lipid research group, poised to participate in new opportunities
- A more efficient analytical pipeline for plant biologists to obtain information on lipid metabolism and lipid-related traits relevant for crop improvement
- A better understanding and improvement of plant growth under biotic and abiotic stress conditions, which scientists can use to improve crop plants by breeding and transgenic approaches
- Knowledge of seed metabolism and biology for improvement of seed oils
- Improvement of crop productivity and quality for human and livestock nutrition, benefiting producers, consumers, and the bio-based economy
Milestones
(2017): Optimized extraction methods for crop plants developed and exchanged among groups Lipid internal standard mixes available for sharing Rapid lipidomic methods for leaves of sorghum, camelina, and soy Survey of lipid molecular species to be analyzed in comprehensive analysis Automated data upload to PMR database Identification of camelina mutants with altered leaf and seed lipid composition Select, clone, and characterize promoter regions for selected oil body coat proteins(2018): Methods for unprecedentedly comprehensive lipidomic analysis of soy, camelina, and sorghum leaf and seed Visualization of lipids in crop plant tissues and localization in plant tissues of lipids not previously visualized Web-based statistical analysis of metabolomics data with transcriptomics data available in PMR PMR linked to other databases Development of a shared database with information about lipid-related genes in crop plants Mapping of genetic lesions affecting camelina mutants with altered leaf and seed lipid composition Development of camelina lines with modified oil composition Develop a strategy for upregulating expression of selected oil body coat proteins
(2019): Large scale growth of camelina lines with modified seed oil composition Generate a transgenic crop line Laboratory test for improved resistance to one or more stresses Evaluate efficacy of oil body protein strategy in transgenic Arabidopsis thaliana
(2020): Extraction and property analysis of modified seed oil Information obtained about the physical and chemical properties of one or more modified seed oils Prepare transgenic soybeans overexpressing selected oil body coat proteins; evaluate changes in lipid composition
(2021): Field trial for improved resistance to one or more stresses
Projected Participation
View Appendix E: ParticipationOutreach Plan
The “LIPIDS of Crops” group will publish its results in scientific journals. Lipidomic and transcriptomic data stemming from the work of the “LIPIDS of Crops” group will be housed in Plant/Eukaryotic and Microbial Systems Resource (metnetdb.org/PMR/). There, lipidomics and transcriptomic data can be viewed by its owners, who can use PMR’s statistical tools for data analysis; the data can made public upon publication of the results. “LIPIDS of Crops” members also will present their results at scientific meetings. Method advances also will be published. Kansas Lipidomics Research Center (KLRC) has a website that will includes extraction and other protocols and information. To enable the adoption of methods in other labs beyond the multistate group, KLRC personnel with also share methods files that drive acquisition of data by a mass spectrometer with labs who own similar instruments. Internal standard mixes will be available for a fee that reflects the cost of the components, their quantification, and assembly. As feasible, lipidomics methods developed during the work of the multistate group will be adapted at KLRC and made available to the scientific public on a fee-for-service basis. KLRC’s fees are designed to cover instrument maintenance, technical support, materials used in analysis, and data processing. Quantitative data are provided in spreadsheets. Members of the multi-state group are active faculty members with postdoctoral trainees, graduate students, and undergraduate researchers in their laboratories. In carrying out the proposed work, we plan to exchange postdocs and/or students among laboratories, with visits ranging from a few days to several weeks, to aid joint projects, to improve student training, and to exchange information and expertise among labs. In addition, Cahoon serves as American Society of Plant Biologists (ASPB) representative for the Midwest Section and Schrick serves as the Midwest ASPB Section’s secretary/treasurer. ASPB Midwest Section meetings are held yearly and will serve as a second meeting place for group members. Students and postdocs from “LIPIDS of Crops” groups will be encouraged to present their work at the meeting. “LIPIDS of Crops” get-togethers at the Midwest ASPB meetings will foster interactions among student and postdoc members of the “LIPIDS of Crops” groups. Some members of the “LIPIDS of Crops” group interact with farmers and students on the subject of GMO crops. Hoffmann-Benning has provided lectures and will again speak to undergraduates majoring in non-science areas at Michigan State University about the generation and use of GMOs. Clemente regularly addresses farmers and public about the advantages of GMOs (e.g., Crossley, 2015).
Organization/Governance
The “LIPIDS of Crops” group will use the Standard Governance for multistate research activities. These include the election of a Chair, a Chair-elect, and a Secretary. All officers will eventually be elected for three-year terms which will be offset by one year to provide continuity. Initial officers will be Basil Nikolau, Chair; Ed Cahoon, Vice Chair; and Jay Thelen, Secretary. At each annual meeting, a new Secretary will be elected, the previous Vice-Chair will become Chair, and the previous Secretary will become Vice-Chair. The Chair will be responsible for organizing the next year’s meeting. Administrative guidance will be provided by an assigned Administrative Advisor and a CSREES Representative.
Literature Cited
Allen DK, Bates PD, Tjellstrom H (2015) Tracking the metabolic pulse of plant lipid production with isotopic labeling and flux analyses: Past, present and future. Prog Lipid Res 58: 97-120 Allen DK, Evans BS, Libourel IG (2014) Analysis of isotopic labeling in peptide fragments by tandem mass spectrometry. PLoS One 9: e91537 Benning UF, Tamot B, Guelette BS, Hoffmann-Benning S (2012) New aspects of phloem-mediated long-distance lipid signaling in plants. Front Plant Sci 3: 53 Bräutigam A, Hoffmann-Benning S, Weber AP (2008) Comparative proteomics of chloroplast envelopes from C3 and C4 plants reveals specific adaptations of the plastid envelope to C4 photosynthesis and candidate proteins required for maintaining C4 metabolite fluxes. Plant Physiol 148: 568-579 Cai Y, Goodman JM, Pyc M, Mullen RT, Dyer JM, Chapman KD (2015) Arabidopsis SEIPIN proteins modulate triacylglycerol accumulation and influence lipid droplet proliferation. Plant Cell 27: 2616-2636 Carmichael RE, Boyce A, Matthewman C, Patron NJ (2015) An introduction to synthetic biology in plant systems: ERASynBio/OpenPlant summer school for early career researchers, September 2014. New Phytol 208: 20-22 Cha S, Zhang H, Ilarslan HI, Wurtele ES, Brachova L, Nikolau BJ, Yeung ES (2008) Direct profiling and imaging of plant metabolites in intact tissues by using colloidal graphite-assisted laser desorption ionization mass spectrometry. Plant J 55: 348-360 Chen M, Mooney BP, Hajduch M, Joshi T, Zhou M, Xu D, Thelen JJ (2009) System analysis of an Arabidopsis mutant altered in de novo fatty acid synthesis reveals diverse changes in seed composition and metabolism. Plant Physiol 150: 27-41 Clemente TE, Cahoon EB (2009) Soybean oil: genetic approaches for modification of functionality and total content. Plant Physiol 151: 1030-1040 Crossley T (2015) Biotechnology: Genetic diversity if the key to crop improvement. Story on a talk by Tom Clemente. Wheat Life. http://wheatlife.org/Issues/01_WLJan15web.pdf Durrett TP, McClosky DD, Tumaney AW, Elzinga DA, Ohlrogge J, Pollard M (2010) A distinct DGAT with sn-3 acetyltransferase activity that synthesizes unusual, reduced-viscosity oils in Euonymus and transgenic seeds. Proc Natl Acad Sci U S A 107: 9464-9469 Fan J, Yan C, Roston R, Shanklin J, Xu C (2014) Arabidopsis lipins, PDAT1 acyltransferase, and SDP1 triacylglycerol lipase synergistically direct fatty acids toward ?-oxidation, thereby maintaining membrane lipid homeostasis. Plant Cell 26: 4119-4134 Fukushima A, Kusano M, Mejia RF, Iwasa M, Kobayashi M, Hayashi N, Watanabe-Takahashi A, Narisawa T, Tohg T, Hur M, Wurtele ES, Nikolau BJ, Saito K (2014) Metabolomic characterization of knock-out mutants in Arabidopsis - Development of a metabolite profiling database for knock-out mutants in Arabidopsis (MeKO). Plant Physiol 165: 948-961 Graef G, LaVallee BJ, Tenopir P, Tat M, Schweiger B, Kinney AJ, Van Gerpen JH, Clemente TE (2009) A high-oleic-acid and low-palmitic-acid soybean: agronomic performance and evaluation as a feedstock for biodiesel. Plant Biotechnol J 7: 411-421 Guelette BS, Benning UF, Hoffmann-Benning S (2012) Identification of lipids and lipid-binding proteins in phloem exudates from Arabidopsis thaliana. J Exp Bot 63: 3603-3616 Guo L, Ma F, Wei F, Fanella B, Allen DK, Wang X (2014) Cytosolic phosphorylating glyceraldehyde-3-phosphate dehydrogenases affect Arabidopsis cellular metabolism and promote seed oil accumulation. Plant Cell 26: 3023-3035 Guo L, Mishra G, Markham JE, Li M, Tawfall A, Welti R, Wang X (2012) Connections between sphingosine kinase and phospholipase D in the abscisic acid signaling pathway in Arabidopsis. J Biol Chem 287: 8286-8296 Hajduch M, Hearne LB, Miernyk JA, Casteel JE, Joshi T, Agrawal GK, Song Z, Zhou M, Xu D, Thelen JJ (2010) Systems analysis of seed filling in Arabidopsis: using general linear modeling to assess concordance of transcript and protein expression. Plant Physiol 152: 2078-2087 Hajduch M, Matusova R, Houston NL, Thelen JJ (2011) Comparative proteomics of seed maturation in oilseeds reveals differences in intermediary metabolism. Proteomics 11: 1619-1629 Hong Y, Devaiah S P, Bahn SC, Thamasandra BN, Li M, Welti R, Wang X (2009) Phospholipase D? and phosphatidic acid enhance Arabidopsis nitrogen signaling and growth. Plant J 58: 376-387 Hong Y, Pan X, Welti R, Wang X (2008) Phospholipase D?3 regulates Arabidopsis response to salinity and water deficits. Plant Cell 20: 803-816 Horn PJ, Korte AR, Neogi PB, Love E, Fuchs J, Strupat K, Borisjuk L, Shulaev V, Lee YJ, Chapman KD (2012) Spatial mapping of lipids at cellular resolution in embryos of cotton. Plant Cell 24: 622-636 Hur M, Campbell AA, Almeida-de-Macedo M, Li L, Jose A, Crispin M, Nikolau BJ, Wurtele ES (2013) A global approach to analysis and interpretation of metabolic data for plant natural product discovery. Nat Prod Rep 30: 565-583 Ibrahim A, Schütz AL, Galano JM, Herrfurth C, Feussner K, Durand T, Brodhun F, Feussner I (2011) The alphabet of galactolipids in Arabidopsis thaliana. Front. Plant Sci 2: 95. Jao CY, Nedelcu D, Lopez LV, Samarakoon TN, Welti R, Salic A (2015a) Bioorthogonal probes for imaging sterols in cells. Chembiochem 16: 611-617 Jao CY, Roth M, Welti R, Salic A (2009) Metabolic labeling and direct imaging of choline phospholipids in vivo. Proc Natl Acad Sci U S A 106: 15332-15337 Jao CY, Roth M, Welti R, Salic A (2015b) Biosynthetic labeling and two-color imaging of phospholipids in cells. Chembiochem 16: 472-476 Kilaru A, Tamura P, Isaac G, Welti R, Venables BJ, Seier E, Chapman KD (2012) Lipidomic analysis of N-acylphosphatidylethanolamine molecular species in Arabidopsis suggests feedback regulation by N-acylethanolamines. Planta 236: 809-824 Kim HJ, Silva JE, Iskandarov U, Andersson M, Cahoon RE, Mockaitis K, Cahoon EB (2015a) Structurally divergent lysophosphatidic acid acyltransferases with high selectivity for saturated medium chain fatty acids from Cuphea seeds. Plant J In press Kim HJ, Silva JE, Vu HS, Mockaitis K, Nam JW, Cahoon EB (2015b) Toward production of jet fuel functionality in oilseeds: identification of FatB acyl-acyl carrier protein thioesterases and evaluation of combinatorial expression strategies in Camelina seeds. J Exp Bot 66: 4251-4265 Kimberlin AN, Majumder S, Han G, Chen M, Cahoon RE, Stone JM, Dunn TM, Cahoon EB (2013) Arabidopsis 56-amino acid serine palmitoyltransferase-interacting proteins stimulate sphingolipid synthesis, are essential, and affect mycotoxin sensitivity. Plant Cell 25: 4627-4639 Koo AJ, Cooke TF, Howe GA (2011) Cytochrome P450 CYP94B3 mediates catabolism and inactivation of the plant hormone jasmonoyl-L-isoleucine. Proc Natl Acad Sci USA 108: 9298-9303 Koo AJ, Thireault C, Zemelis S, Poudel AN, Zhang T, Kitaoka N, Brandizzi F, Matsuura H, Howe GA (2014) Endoplasmic reticulum-associated inactivation of the hormone jasmonoyl-L-isoleucine by multiple members of the cytochrome P450 94 family in Arabidopsis. J Biol Chem 289: 29728-29738 Korte AR, Yandeau-Nelson MD, Nikolau BJ, Lee YJ (2015) Subcellular-level resolution MALDI-MS imaging of maize leaf metabolites my MALDI-linear ion trap-Orbitrap mass spectrometer. Anal Bioanal Chem 407: 2301-2309 Lee Y-J, Perdian DC, Song Z, Yeung E, Nikolau N (2012) Use of mass-spectrometry for imaging metabolites in plants. Plant J 70: 81-95 Li L, Hur M, Lee JY, Zhou W, Song Z, Ransom N, Demirkale CY, Nettleton D, Westgate M, Arendsee Z, Iyer V, Shanks J, Nikolau B, Wurtele ES (2015a) A systems biology approach toward understanding seed composition in soybean. BMC Genomics. 16 Suppl 3: S9 Li L, Wurtele ES (2014) The QQS orphan gene of Arabidopsis modulates carbon and nitrogen allocation in soybean. Plant Biotechnol J. 13: 177-187 Li L, Zheng W, Zhu Y, Ye H, Tang B, Arendsee ZW, Jones D, Li R, Ortiz D, Zhao X, Du C, Nettleton D, Scott MP, Salas-Fernandez MG, Yin Y, Wurtele ES (2015b) QQS orphan gene regulates carbon and nitrogen partitioning across species via NF-YC interactions. Proc Natl Acad Sci USA 112: 14734-14739 Li M, Bahn SC, Fan C, Li J, Phan T, Ortiz M, Roth MR, Welti R, Jaworski J, Wang X (2013) Patatin-related phospholipase pPLAIIIdelta increases seed oil content with long-chain fatty acids in Arabidopsis. Plant Physiol 162: 39-51 Li M, Bahn SC, Guo L, Musgrave W, Berg H, Welti R, Wang X (2011) Patatin-related phospholipase pPLAIII?-induced changes in lipid metabolism alter cellulose content and cell elongation in Arabidopsis. Plant Cell 23: 1107-1123 Li M, Baughman E, Roth MR, Han X, Welti R, Wang X (2014a) Quantitative profiling and pattern analysis of triacylglycerol species in Arabidopsis seeds by electrospray ionization mass spectrometry. Plant J 77: 160-172 Li M, Hong Y, Wang X (2009) Phospholipase D- and phosphatidic acid-mediated signaling in plants. Biochim Biophys Acta 1791: 927-935 Li M, Markham JE, Wang X (2014b) Overexpression of patatin-related phospholipase AIIIbeta altered the content and composition of sphingolipids in Arabidopsis. Front Plant Sci 5: 553 Li M, Welti R, Wang X (2006) Arabidopsis phospholipase D?1 modulates defense responses to bacterial and fungal pathogens. Plant Physiol 142: 750-761 Li W, Li M, Zhang W, Welti R, Wang X (2004) The plasma membrane-bound phospholipase D? enhances freezing tolerance in Arabidopsis thaliana. Nature Biotechnol 22: 427-433 Li-Beisson Y, Shorrosh B, Beisson F, Andersson MX, Arondel V, Bates PD, Baud S, Bird D, DeBono A, Durrett TP, Franke RB, Graham IA, Katayama K, Kelly AA, Larson T, Markham JE, Miquel M, Molina I, Nishida I, Rowland O, Samuels L, Schmid KM, Wada H, Welti R, Xu C, Zallot R, Ohlrogge J (2010) Acyl-Lipid Metabolism. Arab. Book: e0133 Liu J, Rice A, McGlew K, Shaw V, Park H, Clemente T, Pollard M, Ohlrogge J, Durrett TP (2015) Metabolic engineering of oilseed crops to produce high levels of novel acetyl glyceride oils with reduced viscosity, freezing point and calorific value. Plant Biotechnol J 13: 858-865 Louis J, Kukula K-L, Singh V, Reese JC, Jander G, Shah, J (2010) Antibiosis against the green peach aphid requires the Arabidopsis thaliana MYZUS PERSICAE INDUCED LIPASE1 gene. Plant J 64: 800-811 Lu C, Napier JA, Clemente TE, Cahoon EB (2011) New frontiers in oilseed biotechnology: meeting the global demand for vegetable oils for food, feed, biofuel, and industrial applications. Curr Opin Biotechnol 22: 252-259 Lu C, Xin Z, Ren Z, Miquel M, Browse J (2009) An enzyme regulating triacylglycerol composition is encoded by the ROD1 gene of Arabidopsis. Proc Natl Acad Sci USA 106: 18837-18842 Lu S, Bahn SC, Qu G, Qin H, Hong Y, Xu Q, Zhou Y, Wang X (2013) Increased expression of phospholipase Dalpha1 in guard cells decreases water loss with improved seed production under drought in Brassica napus. Plant Biotechnol J 11: 380-389 Lu S, Yao S, Wang G, Guo L, Zhou Y, Hong Y, Wang X (2015) Phospholipase De enhances Brasica napus growth and seed production in response to nitrogen availability. Plant Biotechnol J In press Ma F, Jazmin LJ, Young JD, Allen DK (2014) Isotopically nonstationary 13C ?ux Arabidopsis thaliana leaf metabolism due to high light acclimation. Proc Natl Acad Sci USA 111: 16967–16972 Malik MR, Yang W, Patterson N, Tang J, Wellinghoff RL, Preuss ML, Burkitt C, Sharma N, Ji Y, Jez JM, Peoples OP, Jaworski JG, Cahoon EB, Snell KD (2015) Production of high levels of poly-3-hydroxybutyrate in plastids of Camelina sativa seeds. Plant Biotechnol J 13: 675-688 Manandhar-Shrestha K, Tamot B, Pratt EP, Saitie S, Bräutigam A, Weber AP, Hoffmann-Benning S (2013) Comparative proteomics of chloroplasts envelopes from bundle sheath and mesophyll chloroplasts reveals novel membrane proteins with a possible role in C4-related metabolite fluxes and development. Front Plant Sci 4: 65 Markham JE, Jaworski JG (2007) Rapid measurement of sphingolipids from Arabidopsis thaliana by reversed-phase high-performance liquid chromatography coupled to electrospray ionization tandem mass spectrometry. Rapid Commun Mass Spectrom 21: 1304-1314 Marti G, Erb M, Boccard J, Glauser G, Doyen GR,Villard N, Robert CA, Turlings TC, Rudaz S, Wolfender JL (2013) Metabolomics reveals herbivore-induced metabolites of resistance and susceptibility in maize leaves and roots. Plant Cell Environ 36: 621-639 Nakamura Y, Teo NZX, Shui G, Chua CHL, Cheong W-F, Parameswaran S, Koizumi R, Ohta H, Wenk MR, Ito T (2014) Transcriptomic and lipidomic profiles of glycerolipids during Arabidopsis flower development. New Phytol 203: 310-322 Nakata M, Mitsuda N, Herde M, Koo AJ, Moreno JE, Suzuki K, Howe GA, Ohme-Takagi M (2013) A bHLH-type transcription factor, ABA-INDUCIBLE BHLH-TYPE TRANSCRIPTION FACTOR/JA-ASSOCIATED MYC2-LIKE1, acts as a repressor to negatively regulate jasmonate signaling in Arabidopsis. Plant Cell. 25: 1641-1656 Narayanan S, Tamura PJ, Roth MR, Prasad PV, Welti R (2015) Wheat leaf lipids during heat stress: I. High day and night temperatures result in major lipid alterations. Plant Cell Environ In press Narayanan S, Prasad PV, Welti R (2015) Wheat leaf lipids during heat stress: II. Lipids experiencing coordinated metabolism are detected by analysis of lipid co-occurrence. Plant Cell Environ In press Ngaki MN, Louie GV, Philippe RN, Manning G, Pojer F, Bowman ME, Li L, Larsen E, Wurtele ES, Noel JP (2012) Evolution of the chalcone-isomerase fold from fatty-acid binding to stereospecific catalysis. Nature 485: 530-533 Nguyen HT, Park H, Koster KL, Cahoon RE, Shanklin J, Clemente TE, Cahoon EB (2015) Redirection of metabolic flux for high levels of omega-7 monounsaturated fatty acid accumulation in camelina seeds. Plant Biotechnol J 13: 38-50 Nilsson AK, Johansson ON, Fahlberg P, Steinhart F, Gustavsson MB, Ellerström M, Andersson MX (2014) Formation of oxidized phosphatidylinositol and 12-oxo-phytodienoic acid containing acylated phosphatidylglycerol during the hypersensitive response in Arabidopsis. Phytochemistry 101: 65-75 Okazaki Y, Otsuki H, Narisawa T, Kobayashi M, Sawai S, Kamide Y, Kusano M, Aoki T, Hirai MY, Saito K (2013) A new class of plant lipid is essential for protection against phosphorus depletion. Nat Commun 4: 1510 Park H, Graef G, Xu Y, Tenopir P, Clemente TE (2014) Stacking of a stearoyl-ACP thioesterase with a dual-silenced palmitoyl-ACP thioesterase and 12 fatty acid desaturase in transgenic soybean. Plant Biotechnol J 12: 1035-1043 Perera MA, Qin W, Yandeau-Nelson M, Fan L, Dixon P, Nikolau BJ (2010) Biological origins of normal-chain hydrocarbons: a pathway model based on cuticular wax analyses of maize silks. Plant J 64: 618-632 Peters C, Li M, Narasimhan R, Roth M, Welti R, Wang X (2010) Nonspecific phospholipase C NPC4 promotes responses to abscisic acid and tolerance to hyperosmotic stress in Arabidopsis. Plant Cell 22: 2642-2659 Riedelsheimer C, Brotman Y, Méret M, Melchinger AE, Willmitzer L (2013) The maize leaf lipidome shows multilevel genetic control and high predictive value for agronomic traits. Sci Rep. 3: 2479 Roston RL, Gao J, Murcha MW, Whelan J, Benning C (2012) TGD1, -2, and -3 proteins involved in lipid trafficking form ATP-binding cassette (ABC) transporter with multiple substrate-binding proteins. J Biol Chem. 287: 21406-21415 Roston RL, Gao J, Xu C, Benning C (2011) Arabidopsis chloroplast lipid transport protein TGD2 disrupts membranes and is part of a large complex. Plant J 66: 759-769 Roston RL, Wang K, Kuhn LA, Benning C (2014) Structural determinants allowing transferase activity in SENSITIVE TO FREEZING 2, classified as a family I glycosyl hydrolase. J Biol Chem 289: 26089-26106 Samarakoon T, Shiva S, Lowe K, Tamura P, Roth MR, Welti R (2012) Arabidopsis thaliana membrane lipid molecular species and their mass spectral analysis. Meth Mol Biol 918: 179-268 Sanjaya, Miller R, Durrett TP, Kosma DK, Lydic TA, Muthan B, Koo AJ, Bukhman YV, Reid GE, Howe GA, Ohlrogge J, Benning C (2013). Altered lipid composition and enhanced nutritional value of Arabidopsis leaves following introduction of an algal diacylglycerol acyltransferase 2. Plant Cell 25: 677-693. Sarrion-Perdigones A, Palaci J, Granell A, Orzaez D (2014) Design and construction of multigenic constructs for plant biotechnology using the GoldenBraid cloning strategy. Methods Mol Biol 1116: 133-151 Sarrion-Perdigones A, Vazquez-Vilar M, Palaci J, Castelijns B, Forment J, Ziarsolo P, Blanca J, Granell A, Orzaez D (2013) GoldenBraid 2.0: a comprehensive DNA assembly framework for plant synthetic biology. Plant Physiol 162: 1618-1631 Schrick K, Bruno M, Khosla A, Cox PN, Marlatt SA, Roque RA, Nguyen HC, He C, Snyder MP, Singh D, Yadav G (2014) Shared functions of plant and mammalian StAR-related lipid transfer (START) domains in modulating transcription factor activity. BMC Biol 12: 70 Schrick K, Nguyen D, Karlowski WM, Mayer KF (2004) START lipid/sterol-binding domains are amplified in plants and are predominantly associated with homeodomain transcription factors. Genome Biol 5: R41 Schrick K, Shiva S, Arpin J, Delimont N, Isaac G, Tamura P, Welti R (2012) Steryl glucoside and acyl steryl glucoside analysis of Arabidopsis seeds by electrospray ionization tandem mass spectrometry. Lipids 47: 185-193 Smith JM, Leslie ME, Robinson SJ, Korasick DA, Zhang T, Backues SK, Cornish PV, Koo AJ, Bednarek SY, Heese A (2014) Loss of Arabidopsis thaliana Dynamin-Related Protein 2B reveals separation of innate immune signaling pathways. PLoS Pathog 10: e1004578 Smith-Hammond CL, Swatek KN, Johnston ML, Thelen JJ, Miernyk JA (2014) Initial description of the developing soybean seed protein Lys-N(?)-acetylome. J Proteomics 96: 56-66 Stucky DF, Arpin JC, Schrick K (2015) Functional diversification of two UGT80 enzymes required for steryl glucoside synthesis in Arabidopsis. J Exp Bot 66: 189-201. Thelen JJ, Ohlrogge JB (2002) Both antisense and sense expression of biotin carboxyl carrier protein isoform 2 inactivates the plastid acetyl-coenzyme A carboxylase in Arabidopsis thaliana. Plant J 32: 419-431 Troncoso-Ponce MA, Cao X, Yang Z, Ohlrogge JB (2013) Lipid turnover during senescence. Plant Sci 205-206: 13-19 Vu HS, Roston R, Shiva S, Hur M, Wurtele ES, Wang X, Shah J, Welti R (2015) Modifications of membrane lipids in response to wounding of Arabidopsis thaliana leaves. Plant Signal Behav 10: e1056422 Vu HS, Shiva S, Roth MR, Tamura P, Zheng L, Li M, Sarowar S, Honey S, McEllhiney D, Hinkes P, Seib L, Williams TD, Gadbury G, Wang X, Shah J, Welti, R (2014) Lipid changes after leaf wounding in Arabidopsis thaliana: expanded lipidomic data form the basis for lipid co-occurrence analysis. Plant J 80: 728-743 Welti R, Li W, Li M, Sang Y, Biesiada H, Zhou H-E, Rajashekar CB, Williams TD, Wang X (2002) Profiling membrane lipids in plant stress responses. Role of phospholipase D? in freezing-induced lipid changes in Arabidopsis. J Biol Chem 277: 31994-32002 Xin Z, Wang ML, Barkley N, Burow G, Franks C, Pederson G, Burke J (2008) Applying genotyping (TILLING) and phenotyping analyses to elucidate gene function in a chemically induced sorghum mutant population. BMC Plant Biol 8: 103 Xu C, Fan J, Riekhof W, Froehlich JE, Benning C (2003) A permease-like protein involved in ER to thylakoid lipid transfer in Arabidopsis. EMBO J 22: 2370–2379 Yang W, Cahoon RE, Hunter SC, Zhang C, Han J, Borgschulte T, Cahoon EB (2011) Vitamin E biosynthesis: functional characterization of the monocot homogentisate geranylgeranyl transferase. Plant J 65: 206-217. Yang WY, Zheng Y, Bahn SC, Pan XQ, Li MY, Vu HS, Roth MR, Scheu B, Welti R, Hong YY, Wang XM (2012) The patatin-containing phospholipase A pPLAIIalpha modulates oxylipin formation and water loss in Arabidopsis thaliana. Mol Plant 5: 452-460 Yeo YS, Nybo SE, Chittiboyina AG, Weerasooriya AD, Wang YH, Góngora-Castillo E, Vaillancourt B, Buell CR, DellaPenna D, Celiz MD, Jones AD, Wurtele ES, Ransom N, Dudareva N, Shaaban KA, Tibrewal N, Chandra S, Smillie T, Khan IA, Coates RM, Watt DS, Chappell J (2013) Functional identification of valerena-1,10-diene synthase, a terpene synthase catalyzing a unique chemical cascade in the biosynthesis of biologically active sesquiterpenes in Valeriana officinalis. J Biol Chem 288: 3163-3173 Zhang M, Fan J, Taylor DC, Ohlrogge JB (2009) DGAT1 and PDAT1 acyltransferases have overlapping functions in Arabidopsis triacylglycerol biosynthesis and are essential for normal pollen and seed development. Plant Cell 21: 3885-3901 Zhang T, Poudel AN, Jewell JB, Kitaoka N, Staswick P, Matsuura H, Koo AJ (2015) Hormone crosstalk in wound stress response: Wound-inducible amidohydrolases can simultaneously regulate jasmonate and auxin homeostasis in Arabidopsis thaliana. J Exp Bot Accepted Zhao J, Devaiah SP, Wang C, Li M, Welti R, Wang X (2013) Arabidopsis phospholipase Db1 modulates defense responses to bacterial and fungal pathogens. New Phytol 199: 228-240 Zien CA, Wang C, Wang X, Welti R (2001) In-vivo substrates and the contribution of the common phospholipase D, PLD alpha, to wound-induced metabolism of lipids in Arabidopsis. Biochim Biophys Acta 1530: 236-248