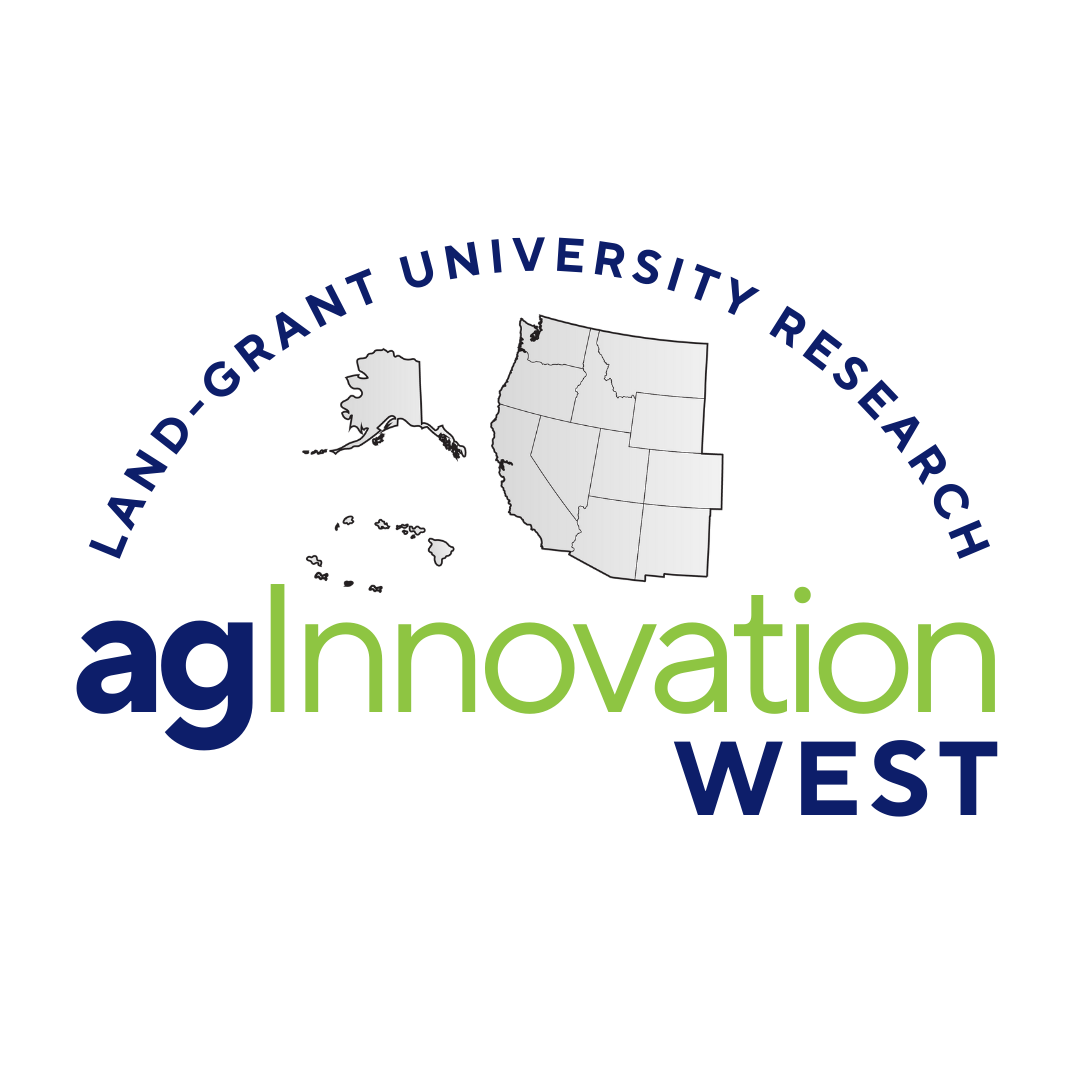
W3171: Germ Cell and Embryo Development and Manipulation for the Improvement of Livestock
(Multistate Research Project)
Status: Inactive/Terminating
W3171: Germ Cell and Embryo Development and Manipulation for the Improvement of Livestock
Duration: 10/01/2014 to 09/30/2019
Administrative Advisor(s):
NIFA Reps:
Non-Technical Summary
Statement of Issues and Justification
At its inception almost 30 years ago, the primary goal of the W171 Regional Research Project (revised and renewed since as project #s W1171 and W2171) was to establish a cooperative, multistate research group comprised of basic and applied scientists that would uncover the mysteries behind germ cell function and embryo development so that these processes could be manipulated for the improvement of livestock. In the three decades since the initiation of this formal research collaboration, significant advances in techniques, technologies, and basic scientific knowledge have been made to this end. Likewise, many of the regulatory and public perception hurdles associated with using genetically modified animals as food and/or fiber sources have been cleared. In fact, forward-thinking investigators (including many active participants in this multi-state project) have produced, to date, at least 23 different genetic modifications to domestic livestock animals to enhance production traits Despite the advances within and outside of this regional research effort, a significant knowledge gap persists regarding the ability to efficiently produce genetic modifications to domesticated livestock species. The efficiencies of these technologies will have to be substantially improved if we are to benefit from the advantages of genetically enhanced farm animals for human food and fiber production. New genome-editing technology is one that will greatly improve the efficiency of genetic engineering but its application in domestic animals has just barely began. Herein, we request to continue pursuit of our research priorities and renew the W-2171 Regional Research Project with the overall goal of producing genetically enhanced animals to improve the efficiency of livestock production systems. The proposed scope of this Regional Research Project falls under both Strategic Goals 1 and 3 of the Strategic Plan for the entire US Department of Agriculture [1], which include mandates to establish and support a competitive agricultural system (including food animal production; Objective 1.3) and to continue the development of agricultural products derived from biotechnological advances (Objective 3.2). Additionally, and to a more specific point, the aims of this research effort are also directly in line with the vision set forth by the National Institute of Food and Agriculture (NIFA) Strategic Plan (Objective 2.2 of Strategic Goal 2) which promises to provide research, education, and extension to increase the efficiency of agricultural production and marketing systems. The labors of the Project members toward these goals and objectives have been and will continue to be evaluated according to the following performance criteria: 2.2.7 - Increase and improve the reproductive performance of animals (NIFA Knowledge Area 301); 2.2.9 - Develop and apply information and technology for genetic improvement of animals (NIFA Knowledge Area 303); and 2.2.11 - Improve understanding of fundamental animal physiological processes (NIFA Knowledge Area 305). In addition, research to increase the practicality of making genetically enhanced livestock animals is directly in line with the Food Animal Integrated Research (FAIR) 2012 Focus Area 1 [2]. Key topic 1-4 under this goal is to enhance livestock animal reproductive efficiency, including through assisted reproductive technologies. Key Topic 1-3 Goal 5 (Connecting -omics to animal production) is also relevant to the research proposed under this Project. Beneficiaries of this multistate research endeavor include: 1) individual livestock producers in the Western states, as well as farmers and ranchers across the country; 2) rural communities of the West; 3) consumers of animal products within the Western region, U.S. and the world; and 4) the scientific community worldwide. Livestock producers will benefit from increased profits as a result of reduced input costs linked to efficient production systems, improved performance of animals, and value-added products. The economic stimulus afforded to a rural community that is located near a profitable and sustainable animal industry can be dramatic, providing many opportunities otherwise unavailable to its residents and enhancing the quality of life. Consumers will be impacted by reduced food prices associated with increased efficiency of livestock production, meat, dairy, and/or other food products with enhanced health benefits, an improved environment resulting from livestock systems producing less waste, and the availability of food sources to meet the demands of an ever increasing population at both the national and international level. Consumers can also benefit from genetically engineered livestock that are resistant to diseases, permitting the use of less/no antibiotics in animal feed. Investigators within the scientific community will also benefit from the efforts of the Project members. The use of gene transfer alone or in combination with somatic cell nuclear transfer is very useful for obtaining a variety of experimental information. Some examples are insight into the cell cycle, nuclear and cytoplasmic programming or reprogramming, genomic imprinting, gene expression, epigenetics and developmental processes. This information can be used in studies to examine basic biological, biomedical, genetic and evolutionary questions, in addition to agriculture applications. The economic significance of genetically enhanced animals to U.S. animal agriculture in the future cannot be estimated with any confidence. However, the livestock and dairy industries within the U.S. generated over 170 billion dollars of on-farm receipts in 2012 [3], and any increases in animal production efficiencies would be extremely impactful within the Western region as well as nationally. Within the states comprising this regional research project, livestock numbers (as of January 1, 2012) included 28.9 million head of beef cattle, 43.7 million swine, 2 million sheep, and 3.3 million dairy cows (that produced 76.5 billion pounds of milk in 2012); the total value of livestock, poultry and their products within these states was $68.4 billion [4]! Therefore, even an incremental 1% increase in the cumulative value of these animals (or a corresponding decrease in the production costs) would inject an additional $680 million dollars into these local economies! Notably, these numbers do not include the tangible and intangible monetary considerations associated with the burgeoning market for large transgenic animals in biomedical research. Even small increases in the efficiency of transgenic animal production will repay research costs many times over. Production of genetically enhanced animals for the production of food and fiber holds significant promise for consumers, animal producers, and scientists, their respective communities, and our environment as well. Somatic cell nuclear transfer (SCNT) or cloning, has dramatically advanced animal biotechnology, and significantly enhanced our ability to produce genetically altered livestock. This technology has three broad applications: 1) applied animal breeding to propagate animals with superior quantitative traits and/or pedigrees (prime grade steers, proven dairy bulls, e.g.); 2) a tool for basic research, (i.e., mechanisms of cellular differentiation and dedifferentiation), and 3) a biotechnological tool (more efficient approaches to produce genetically enhanced farm animals). The combination of SCNT technology with genetic modifications of somatic cells has resulted in dramatic advancements in the production of genetically modified animals. This has opened new avenues to produce livestock with improved carcass characteristics, that yield a leaner, more desirable meat, with increased disease resistance, and that are more efficient in growth, reproduction, and wool or milk production [5]. Specific examples of genetically enhanced animals with application to the livestock industry include: 1) swine that produce omega-3 fatty acids in their meat, enhancing its health benefits [6]; 2) disease resistant dairy cows (i.e., mastitis, Mad-cow disease) that require less pharmaceutical intervention to produce high quality, safe milk products [7]; 3) sows that lactate milk containing human lysozyme proteins, improving piglet growth and survival [8]; 4) chickens that are resistant to avian influenza, thus improving on-farm animal health and welfare and providing safer and more consumer-friendly poultry products [9]; and 5) males of livestock species that produce mono-sex sperm and therefore, sex-specific progeny [10]. Increased efficiencies in production of animal foodstuffs can be of economic benefit to both consumers and producers. In addition, more efficient production of food and fiber has obvious advantages to the environment in terms of reduced use of natural resources. Consistent with this, investigators have developed swine that produce phytase, an enzyme that breaks down phosphorous, in their saliva, reducing emissions in manure that may be hazardous to the environment [11]. Finally, construction of genetically enhanced livestock animals for use in human biomedicine has also developed considerably [12, 13]. In addition to genetically enhanced animals that serve as models for human biology and disease, the potential for harvest of pharmaceutical proteins, antibodies and tissues/organs from these animals for specific medical applications is certainly impactful [14]. Examples of genetically enhanced livestock with significance to human medicine include pigs modified to aid in transplantation of their organs into humans [15, 16], goats engineered to produce human blood coagulation factors in their milk [17] and cattle that produce human antibodies [18-20]. To offset the costs associated with these technologies, development of a nucleus herd of genetically enhanced livestock for implementation into production systems would need to offer a large potential benefit [21]. However, it is not difficult to imagine a cryo-bank of cloned embryos with genetic enhancements available for direct purchase by producers. Even without implementation of genetic modifications, producers could significantly improve the average performance of their animals in a single generation, progress that is unmatched in traditional breeding programs [21]. The current market for products from genetically modified livestock animals is dampened by regulatory and consumer-driven concerns, yet incremental progress is being made to overcome these hurdles. The sale and clinical use of a drug produced by transgenic animals was first approved in Europe in 2006 [14], and subsequently in the US in 2009 (Reuters). This product (ATryn®; GTC Biotherapeutics) is utilized for the prophylaxis of venous thromboembolism in surgery of patients with congenital anti-thrombin deficiency. Also in 2006, the US Food and Drug Administration (FDA) released a comprehensive 678-page report that concluded that food products from animals cloned using SCNT were indistinguishable from those harvested from non-cloned animals, and in 2008 approved the release of cloned animals produced by standard NT methods (i.e., no gene targeting) to be marketed without special labeling. Members of this regional project such as those in Connecticut and other stations provided pivotal data to this assessment. One experiment station (IL) within the W-2171 Regional Research Project verified the absence of a transgene in control animals demonstrating the lack of transmission after co-habitation and post-mating with transgenic animals, providing a critical first step toward rigorous scientific data for risk assessment of transgenic livestock [22]. These findings have been corroborated by others in different circumstances [23]. As a next logical step, legal and scientific mechanisms have recently been drafted to market a genetically enhanced livestock animal (M.B. Wheeler, personal communication), representing a significant advancement for the field of animal biotechnology. Therefore, translation of science performed by members of the Project to livestock producers is already in progress, marking a major impact of research performed by the W-2171 group. Current procedures for the production of experimental animals with genetic enhancements can involve the use of in vitro oocyte maturation (IVM), in vitro fertilization (IVF), in vitro culture (IVC), cell culture and NT either before or after gene transfer. Combined, these technologies are inefficient, so before genetically enhanced animals can contribute significantly to livestock production systems, construction of these animals will have to be far more efficient. The inefficiencies occur at many levels including in vitro production (IVP) of embryos, nuclear transfer, and establishment of pregnancy. The use of in vitro-derived embryos is much more practical than recovery of in vivo-derived embryos, but IVM, IVF and IVC methodologies remain suboptimal. In the bovine system, blastocyst production by in vitro methods has plateaued at around 40 % despite various attempts to improve culture conditions, falling short of the 85 to 95% development rate that occurs in vivo. And similarly disappointing results can be observed using the swine model, where only 30-40% of IVP embryos cultured in vitro will develop into blastocysts. Carefully controlled studies in model organisms have shown that development of in vitro produced (IVP) embryos following transfer into surrogate recipients is substantially poorer compared to in vivo produced (IVV) embryos [24-28]. Moreover, the quality of IVP embryos, by virtually every embryo quality metric, is much more variable than IVV embryos [26, 27]. Embryo culture media formulations are generally very good at supporting embryo development to the blastocyst stage [29-31]. However, it is also abundantly clear that in vitro manipulations during early development can alter gene expression [32-36] and the epigenetic control thereof [37-43] in pre-, peri- and post-implantation IVP embryos, and that these alterations can even persist into postnatal life. Although we continue to make significant advances in NT technology for livestock and laboratory species [44-46], much is still to be learned regarding the biology and application of these methods to produce genetically enhanced animals. Cloning by somatic cell nuclear transfer continues to be inefficient, with current success rates averaging 1-10%, depending on species [47-49]. In addition to the inefficiencies associated with the production of genetically enhanced animals, the methodologies are costly, highly time consuming and labor intensive. Up to 10 hours of labor may be required to produce a single cloned bovine embryo for transfer into a recipient female. When this is coupled with a 1-5% pregnancy rate, an estimated 1,000 hours are required to produce a single transgenic offspring. Clearly, this technology remains relatively inefficient at present and needs improvement before it will be widely adopted into mainstream livestock animal production systems. Members of this multi-state research project are actively pursuing the techniques and the knowledge that will improve the efficiency of producing transgenic livestock animals. These research pursuits include (but are not limited to) the following areas of concern: • A basic understanding of the mechanisms of normal gamete and embryo function are necessary before any meaningful diagnosis of faulty embryogenesis is possible. • Nuances of oocyte and/or donor cell physiology and the responses of these tissues to their respective environments may have profound effects on the success rates of SCNT. In isolated experiments, cloning success rates of 20-40% have been reported [47]! An appreciation for the circumstances surrounding such successes could result in widespread changes to donor cell, oocyte, or embryo culture protocols that might make survival rates of 30% the norm rather than the exception. Understanding of epigenetic changes in both the somatic donor cell and the cloned embryos during SCNT is very actively perused by members of this project and will continue to be a major area of study. • The inefficiencies associated with production and selection of genetically modified somatic cells for use as karyoplast donors in SCNT also contribute to the lower success rates of cloning [50, 51]. Incremental progress has been made towards improving the efficiencies of this aspect of SCNT with the adaptation of viral delivery systems (i.e., retroviruses, adenoviruses and lentiviruses) for the production of transgenic donor cells and for the direct virus-mediated transformation of embryo cells [52-54]. The advent of a new generation of genome editing tools, including zinc finger nucleases (ZFNs), transcription activator-like effector nucleases (TALENs), and clustered regulatory interspaced short palindromic repeat (CRISPR) /Cas-based RNA-guided DNA endonucleases promises to revolutionize this process even further [55]. It should be noted however, that these delivery systems only improve the efficiency of gene transfer, but have little impact on the inefficiencies associated with IVM, IVF and IVC and SCNT procedures. • Placental defects are a key factor in the low embryonic, fetal, and neonatal survival rates after SCNT in all species studied to date Moreover, alterations in placental physiology due to embryo culture can result in large offspring syndrome and have long-tern consequences to offspring heath. A more thorough understanding of the differentiation and function of trophoblast and other placental cells in normal and abnormal embryonic development is needed before the necessary and appropriate steps of intervention can be undertaken to ensure more successful development. • Finally, short and long-term storage of genetically altered embryos is necessary for efficient production of transgenic animals but cryopreservation of manipulated embryos needs to be investigated and improved as well [56]. A recent report from the National Institute of Food and Agriculture affirms the following: “Reproductive success in livestock is essential for the economic livelihood of producers and ultimately affects the consumer cost of meat and other animal products. In many livestock production systems, poor fertility is a major factor that limits productivity. The ability of animals to reproduce efficiently is an integral component of animal agriculture. However, infertility is a problem to some degree in all animal production systems, including aquaculture species. Reproductive failure is one of the most significant factors that limit the productivity of animal production systems and result in millions of dollars in lost profits annually. A major challenge facing many producers is finding practical, cost-effective ways to improve reproductive performance without compromising the production of safe, high quality meat and milk products [57].” Thus, in considering these and other knowledge gaps and critical needs within the fields of production agriculture and biomedical modeling, it is evident that consequences of not addressing basic questions of reproductive efficiency – including the production of transgenic livestock animals – are: • continued reproductive inefficiencies at all levels and in all segments of animal agriculture; • the collective losses of millions of dollars in opportunity costs associated with reproductive inefficiency (according to reference [58], the US dairy industry alone loses between $424 million and $2.88 billion to poor reproductive performance annually!); • an inability to supply the world’s growing population (world population predicted to swell to almost 10 billion by 2050; see reference [ 59]) with high quality animal protein they need and want using ever-less arable land; and • a compromised ability to appropriately model human health concerns using genetic or other large animal models of human disease. Investigation of challenging questions can be achieved very efficiently via a multistate research project of this nature. The combined expertise and resources of member scientists and institutions from both within the Western region as well as stations residing outside of the region can be utilized. Another advantage to the regional research model is that alternative approaches can be examined in multiple laboratories and the effective procedures further tested in the remaining laboratories. Oocyte and embryo procedures appear particularly laboratory dependent; for example, the optimal exposure time for vitrification of mouse oocytes and mouse blastocysts varied significantly among laboratories [60-62]. Examination of epigenetic alterations in NT-derived embryos compared to in vivo-derived embryos, improvements in NT methods and the development of embryonic/somatic cell lines to serve as nuclear donors are other areas that would benefit from this multiple laboratory approach. Isolation of pluripotent stem cells for agricultural species has been challenging and as yet, has not been fully successful. Sharing of information and approaches across this multi-state project is critical in advancing stem cell biology and its application to farm animals. This renewal proposal will evaluate two critically important areas to the future success of animal biotechnology: 1) understand the biology and underlying mechanisms of gamete development, fertilization, and embryogenesis; and 2) refine methods for production of genetically enhanced animals to improve livestock production efficiency.
Related, Current and Previous Work
As outlined in the Statement of Issues and Justification, understanding the underlying biological mechanisms associated with gamete development and preservation are key for improved embryo production systems. In the following subsections, we provide a brief review of specific areas related to our Project objectives. Oocyte Maturation Successful oocyte maturation is dependent on two events, nuclear and cytoplasmic maturation [63]. Within the oocyte, levels of cAMP [see Eppig JJ in 64, 65, 66] and a Gs-coupled receptor [67, 68] regulate meiotic arrest. Characteristics of the meiotic spindle can be used to evaluate oocyte quality [69, 70]. However, the process of nuclear maturation occurs spontaneously upon removal of the cumulus-oocyte complex (COC) from the follicle and does not represent a significant problem using established culture conditions. In contrast, the process of cytoplasmic maturation appears to be the critical factor that determines the success of subsequent embryo development. Determining the indicators of cytoplasmic maturation in the oocyte would enable more efficient selection of oocytes for IVF and IVP. Currently, there is no defined method of measuring cytoplasmic maturation other than successful fertilization and embryo development [71, 72]. Although much more research is required, several cellular and molecular predictors of oocyte quality show promise [73-75] including inadequate distribution of mitochondria [76, 77], mitochondrial DNA deletions [78, 79] and reduced glucose-6-phophate dehydrogenase levels [80]. Fertilization Successful fertilization in mammals is dependant on a number of factors including meiotic/morphological changes during spermatogenesis, acquisition of epidydymal/seminal plasma proteins, attainment of motility, capacitation, binding of acrosome-intact sperm to the zona pellucida of the oocyte, acrosome reaction, fusion of the acrosome-reacted sperm with the oocyte plasma membrane, and oocyte activation [81-83]. Although the use of IVF to produce farm animal embryos has become routine, subsequent embryonic development in vitro remains far below that of in vivo embryos, suggesting that more research is required to optimize these factors. Despite some progress in identification of spermatozoa proteins playing a critical role in fertilization, more research is required to determine the underlying functional mechanisms associated with these proteins [84, 85]. As the oocyte matures, the protein composition of the plasma membrane changes including an increased ability to bind and/or fuse with sperm. Integrins located on the plasma membrane of bovine oocytes have been implicated in both fertilization and oocyte activation [86]. Embryo Development Early embryogenesis is regulated by developmentally controlled events including proper changes in transcriptional machinery and activation of embryonic genes to support further development [87, 88]. It is important to improve IVC systems in order to produce embryos with high developmental competence for use in agricultural and biomedical research, as well as animal biotechnology [89-91]. Although the methodology for maintaining embryos from livestock in culture has existed for many years, the ability of the present systems to support normal development is limited. Studies have been performed to remove, replace or delay the addition of serum into the culture systems [92]. However, the results indicated that removal of serum from the culture medium does not always prevent the occurrence of large offspring syndrome [93], suggesting other causes of this severe side effect of IVP. A number of different types of semi-defined and defined media have been designed utilizing a variety of supplements [94-97]. The primary problem associated with current culture systems is that they do not mimic the changing oviductal/uterine environment. Microfluidics and small mechanical systems were created a means for dynamic culture on a volumetric scale and more consistent with the needs of the embryo [98]. Even with those improvements, lack of biologic culture conditions necessitates further innovation in tissue culture methodology and further research in this area. Epigenetics Methylation of DNA and histone modifications (methylation, phosphorylation and acetylation), has become a primary area of focus during early embryonic development [99-102]. Shortly after fertilization, mammalian embryos undergo genome-wide epigenetic reprogramming by demethylation, followed later by de novo remethylation [103]. This epigenetic reprogramming is essential for normal development to proceed. Toward this end, aberrant reprogramming has been clearly linked to failed embryonic development [103-105]. Moreover, inadequate epigenetic reprogramming during early mammalian development may result in LOS and other developmental abnormalities [100, 106, 107]. Thus, understanding the factors and mechanisms, which control epigenetic reprogramming during early mammalian development is of critical importance. Nuclear Transfer With significant advancements in the area of SCNT [108], many experimental stations have evaluated the procedures and underlying biology associated with SCNT. Although scientists can successfully reprogram somatic cells prior to NT and produce live animals, many questions remain regarding the inefficiency of the process, the molecular and cellular effects reprogramming has on somatic cells subsequently used as karyoplasts, and the impact of SCNT on the health of resultant clones. In NT, the somatic nucleus has to be reprogrammed in order to restart and continue the developmental process. This requires that tissue-specific genes be inactivated and embryo-specific genes necessary for normal embryo development re-activated within the donor nucleus [105, 109]. The factors affecting the efficiency of NT are: enucleation of the recipient oocyte, fusion of the transplanted nucleus to the enucleated oocyte cytosol, activation of the oocyte, and "reprogramming" of the transferred nucleus [110, 111]. Surprisingly, there is evidence of a significant degree of reprogramming at the gene expression level in cloned preimplantation embryos compared to their IVF counterparts [105, 110-112]. However, evidence seems to suggest that most of the incomplete reprogramming is related to epigenetics [105], an area of research that has moved to the forefront of science. The general consensus of those in the field is that nuclear transfer efficiency is much too low (< 10%) to be economically viable except in very limited applications. Any increase in efficiency will greatly enhance the value of this technology and contribute to our understanding of changes that must occur in chromosomes to allow appropriate embryonic gene expression patterns. It is also critical to understand how potentially subtle modifications in the nuclear structure impact the ability of a cell to contribute to production of offspring. Despite the challenges, nuclear transfer offers unique opportunities in both agriculture and biomedical fields as well as in basic research [113-115]. Sex Determination The ability to pre-select the sex of offspring would have tremendous economic benefit to livestock producers. One approach has been to physically separate X- from Y-bearing spermatozoa [116]. To date, modified flow cytometry is the only separation procedure that has been successful in sorting X- from Y-bearing spermatozoa [117]. Although new methods are being explored, flow cytometry remains the most efficient method to sort semen. While flow cytometry has been improved as evidenced by success with frozen-thawed, sex-sorted and refrozen-thawed ram spermatozoa [118], the high cost of equipment and complexity of the procedure remains a limitation to widespread application. Alternative methods of sex determination include ultrasound of the fetus [119], amplification of fetal DNA from maternal plasma [120], oligonucleotide microarray [121] or RNA-seq [122] of embryos. Cryopreservation In vivo produced embryo cryopreservation has been quite successful [123-125]. Alterations in IVM and IVC can affect the susceptibility of in vitro-produced embryos to cryo-damage. With increased utilization of embryo manipulation procedures, it is critical that we gain a better understanding of the impact these technologies have on successful cryopreservation and develop strategies to prevent the occurrence of detrimental alterations to embryos exposed to manipulation [56]. Reduction of the cytoplasmic lipid content of bovine embryos with phenazine ethosulfate (PES) improved cyrotolerance [126]. Furthermore, advances in vitrification have allowed for successful cryopreservation of goat [125], porcine [127], equine [128], ovine [129] and bovine embryos [130]. Successful vitrification of porcine [131], equine and bovine oocytes [132] has been achieved. Mature oocytes survived vitrification better than immature oocytes. Stem Cell Biology Despite the historic success with isolation and subsequent gene targeting of mouse ES cells, isolation of pluripotent stem cells for agricultural species has been extremely challenging [133]. This lack of success has been offset by dramatic improvements in SCNT, potentially bypassing the need for ES cells. However, as described in the Nuclear Transfer subsection, many difficulties associated with SCNT remain to be solved, especially the inefficiency of the process. Another concern with SCNT is the ability to maintain somatic cells in culture long enough for successful gene targeting and subsequent selection [134]. Multiple laboratories have characterized ES-like or EG (derived from primordial germ) cells for livestock animals [21, 135-137], however, germline chimerism has not been established [138]. More recently, investigators have focused on the isolation of stem cells from a multitude of tissue sources such as adipose, bone marrow, and testis [88, 139, 140]. Induced pluripotent stem cells (iPSC) in which somatic cells are reprogrammed using pluripotency-associated transcription factors may provide an attractive alternate approach, as demonstrated in pigs [141, 142]. In addition, the potential of ES and iPS cells to differentiate into germ cells represents an important germ cell source for production of genetically enhanced animals [141]. Duplication A search of CRIS and NIMSS suggests that other multistate research groups have a similar purpose of improving reproductive efficiency of livestock [(NC1201): Methods to Increase Reproductive Efficiency in Cattle; (NCERA_TEMP057): Swine Reproductive Physiology; (S1061): Nutritional Systems for Swine to Increase Reproductive Efficiency; (NE1227): Ovarian Influences on Reproductive Success in Ruminants; and (S_TEMP3302): Genetic Improvement of Adaptation and Reproduction to Enhance Sustainability of Cow-calf Production in the Southern United States; and (W2112): Reproductive Performance in Domestic Ruminants]. Of those projects, only NE1227 uses similar approaches for evaluation of reproductive success. However, that project does not delve into developmental mechanisms and assisted reproductive technologies. Given the very specific focus of the W_TEMP 3171 group (namely, gamete biology, early embryo development and transgenic technologies for livestock animals), the currently proposed work does not duplicate effort with any other multi-state research project.
Objectives
-
Understand the biology and underlying mechanisms of gamete development, fertilization, and embryogenesis.
-
Refine methods for production of genetically enhanced animals to improve livestock production efficiency.
Methods
Objective 1: Understand the biology and underlying mechanisms of gamete development, fertilization and embryogenesis. Overall aim of this research area is to gain a better understanding of the biological requirements for successful gamete maturation, fertilization, and subsequent embryonic development. The production of live offspring is dependant upon all of those events occurring in a well-orchestrated fashion. More importantly, perhaps, is the fact that a better understanding of the mechanisms underlying these biological processes (i.e., epigenetics) will lead to the production of more healthy, viable offspring. This, in turn, will improve many of the in vitro systems even further, advancing them yet closer to conditions present in vivo. A number of member experiment stations will be investigating this objective including: AR, CA, CT, CO, IL, IN, LA, MD, MS, NC, NE, NV, OR, SC, UT, WV. Oocyte Maturation and Developmental Competence This area of investigation has been and will continue as a strong collaborative subgroup of this multistate research project (CA, CT, CO, IL, LA, MS, NC, NE, NV, WV, UT). Goal of this subgroup will be to investigate the factors associated with and necessary for oocyte development. The efficiency of IVM systems is still sub-optimal. A better understanding of the molecular and cellular mechanisms underlying oocyte maturation will provide information that will help increase the efficiency of IVM procedures. One focus area of the group will be regulation of nuclear and cytoplasmic maturation in domestic species. Although questions regarding the molecular mechanisms underlying nuclear maturation remain, nuclear maturation does not present a significant problem with current IVM culture systems. However, there is no defined method of measuring cytoplasmic maturation other than successful fertilization and embryo development [71]. This group will evaluate changes that occur during cytoplasmic maturation using standard IVM protocols for respective livestock species in an attempt to isolate specific predictive indicators of mature oocyte cytoplasm. Similarly, a better understanding of the factors and mechanisms underlying oocyte maturation will lead to the isolation of biomarkers for the ability of the oocyte to fertilize and develop as an embryo, or developmental competence. Although intensively investigated, there is a gap in knowledge regarding markers of developmental competence. Researchers will identify markers for developmental competence of oocytes in livestock species by: 1) comparing IVM-derived vs. in vivo-derived oocytes; 2) examination of oocytes from more compared to less mature females (i.e., sow vs. gilts or pubertal vs. pre-pubertal); 3) determining differences between oocytes exposed to hormones/growth factors/supplements known to enhance development either in vivo or in vitro in comparison with controls and heat stress conditions; and 4) utilizing genetic strains of animals that are divergent for quality and/or rates of oocyte/embryo development. The search for markers of developmental competence will utilize functional genomic (microarray, RNA-Seq), proteomic (immunofluorescence, immunocytochemistry, stains/dyes for cellular organelles and viability, western blots, protein arrays, mass spectrometry), metabolomics, and other molecular (chromatin immunoprecipitation and small RNA protocols) approaches. In addition, next generation sequencing procedures, which allow high-throughput analysis of the whole genome, will be employed. This technology will allow for easier identification of genetic markers, or single nucleotide polymorphisms (SNPs), that might be linked to developmental competence of the oocyte. Sperm Physiology This workgroup (AR, CA, CO, LA, MS, SC) will evaluate the biology associated with sperm development including meiotic and morphological changes. Methods to increase production of fertile sperm are critical to improving the efficiency of IVF procedures and subsequent embryonic development and will directly benefit livestock production as well. Livestock males will be evaluated for fertility measures including CASA analysis of semen samples and standard staining protocols. Similar to the Oocyte Maturation and Developmental Competence group, this subgroup will utilize functional genomic approaches to identify markers of fertility in bulls/boars and implement these into functional genetic tests to predict fertility/infertility in males during normal and heat stress conditions. Genetic markers will be correlated to measures of fertility from the same animals, as well as with cleavage rates and embryo development after fertilization of oocytes using IVF, intracytoplasmic sperm injection (ICSI) or natural fertilization. Fertilization Mechanisms The cellular and molecular aspects of fertilization and oocyte activation will be explored by this group (CA, CO, IL, MS, NC, NV, UT, WV). One mechanism identified by this group for further investigation is the regulation of polyspermy. Although its importance may vary between species, polyspermy remains a significant difficulty in successful porcine IVF procedures. Researchers will try to isolate sperm/oocyte factors as well as conditions that may contribute to polyspermic conditions. Similarly, the group is also interested in examining basic mechanisms of fertilization in domestic livestock species. Despite some progress in identification of spermatozoa proteins playing a critical role in fertilization, more research is required to determine the underlying functional mechanisms associated with these proteins [84]. Collaborative efforts of the group will continue to isolate fertilization-specific proteins, including analysis of sperm protein-22 in equine spermatozoa, using proteomic approaches. As the oocyte matures, the plasma membrane increasingly gains the ability to bind and/or fuse with sperm. Integrins located on the plasma membrane of bovine oocytes have been implicated in both fertilization and oocyte activation [86]. Two stations (CO, UT) linked the ?V and ?1 subunits of integrins, focal adhesion kinase and Src family kinases, as well as IP3, to fertilization and activation of bovine oocytes. The group will investigate downstream intracellular signaling intermediates specific to these cellular pathways that are activated within the oocyte during fertilization. In the pig, downstream pathways associated with two putative oocyte membrane receptors for sperm, shown to be markers of oocyte fertilizability and reduced by heat stress, will be examined utilizing established protocols that incorporate specific antibodies directed against these factors. Early Embryonic Development Another large collaborative workgroup (AR, CA, CO, CT, IL, IN, MD, NC, NE, OR, WV, UT), these scientists will investigate factors underlying the early stages of embryonic development. One major area of collaboration will be to optimize methods for performing microarray and RT-PCR array analyses on embryos at early stages of development as well as analysis of single embryos from mice, cattle and pigs. The CT and CA station will provide vast expertise with microarray as well as RNA-seq analysis of embryos. Other stations will collect embryos from specific experimental designs to isolate factors vital to these early developmental pathways and send them to CT and CA for microarray/RNA-seq studies and subsequent statistical analyses. Specifically, some investigators will focus on genetic regulation of the maternal to zygotic transition in livestock species. Identification of factors influencing this critical step in preimplantation development will greatly enhance our ability to optimize IVC systems, enhancing the competence of embryos. Shortly after fertilization, mammalian embryos undergo genome-wide epigenetic reprogramming by demethylation, followed later by de novo remethylation [103]. Of primary interest, the effects of epigenetics at this extremely important stage of development will be targeted. Additional studies will pursue peri- and post-implantation development, including genetic regulation of conceptus and placental development. The MD station has initiated a study using metabolomics and fluxomics to investigate the impact of energy sources on early embryonic development. The CO station has ongoing studies focusing on the molecular and hormonal regulation of placental development and differentiation that is absolutely critical for establishment of pregnancy and embryo survival. This is important as impaired placental development can lead to conditions such as intrauterine growth restriction, which in turn have long-term consequences postnatally. In Vitro Embryo Manipulation This workgroup (CA, CO, CT, IL, LA, NC, UT) will examine the effects of in vitro manipulation procedures on both peri- and post-implantation development, especially the effect of embryo manipulation on gene expression during development. As suggested below, embryos that are manipulated in vitro are much more difficult to freeze than in vivo-derived embryos. In a similar vein, this group will determine the impacts of embryo manipulation on global gene expression patterns and epigenetics as these embryos continue development leading up to, during and after implantation, including effects during fetal development (i.e., intrauterine growth restriction). Objective 2: Refine methods for production of genetically enhanced animals to improve livestock production efficiency. Overall aim of this research objective is to enhance the success of each step in the embryo micromanipulation process that is required for successful production of genetically enhanced livestock animals. Continual advances in gene transfer techniques and NT methodology have led to a crucial need for more research that will lead to increased incorporation of these methods into livestock production systems. Research under Objective 2 will be conducted by multiple research institutions comprising the Project: AR, CA, CO, CT, GA, IA, IL, IN, LA, MD, MS, MT, NC, NE, OR, UT, WA. Transgenic Animals This workgroup (CT, IL, LA, MD, NE, UT) will work together toward the production of transgenic animals with agricultural applications, exploiting siRNA gene knock-down technologies. Investigators at NE are studying a form of the gonadotropin-releasing hormone (GnRH) receptor (GnRHR), termed the GnRHR II. This 5- and/or 7-transmembrane receptor is expressed ubiquitously and likely has a strikingly different function than the historic GnRHR, now termed GnRHR I, whose primary functional site is gonadotrope cells within the anterior pituitary gland. Uniquely, the GnRHR II gene is absent in mice and rats; therefore, the pig represents a primary model to determine the in vivo function of this novel G-protein-coupled receptor. Various siRNA oligonucleotides will be tested in a swine testis cell line to determine the siRNA with the strongest ability to reduce the production of GnRHR II. Next, lentiviral vectors will be constructed that contain a constitutively active promoter fused to the selected siRNA and viral particles will produced. Viral particles will be sent to stations with micromanipulation expertise (CT, IL, LA, MD) for injection of particles within the perivitelline space. Embryos will either be transferred into recipient females or rapidly returned to the NE station for subsequent transfer into surrogate mothers. We anticipate that a collaborative experiment of this nature could eventually lead to a team of highly trained scientists with expertise in the production of siRNA knock-down livestock animals. Both anticipated increases in efficiency of gene delivery and simpler microinjection procedures will likely lead to accelerated use of this technology. This group will also pursue the production transgenics with his-tag constructs, development of a mesenchymal stem cell knock-out animal, and production of genetically enhanced animals that would address environmental concerns such as CO2 fixation in ruminants. Recent experiments (CO) have been conducted utilizing transgenic approaches to study gene function specifically in the trophectoderm/trophoblast in livestock animals. Continued development of this approach provides a powerful tool to generate gene “knock-out” and “knock-in” models of early placental development, and yield much insight into the critical events necessary for establishment of pregnancy. One of the projects that UT group is working on is introduction of Callipyge mutation into the goat genome. A novel TALEN (Transcription Activator-Like Effector Nuclease) mediated genome-editing approach is used to move important agricultural traits to a new genetic background (different species). Precise genome editing is based on the ability of engineered nucleases (eg., TALENs) to cut a targeted position in the genome, then a double-stranded break stimulates either homologous recombination or non-homologous end-joining mutagenic repair, which could introduce a targeted mutation into a specific genomic location [143, 144]. The use of this novel TALEN mediated genome-editing approach combined with somatic cell nuclear transfer (SCNT or cloning) will allow moving a single base mutation from one species to another. This study is focused on the introduction of the Callipyge (CLPG) mutation into the goat genome. CLPG is a naturally occurring mutation in sheep, which is characterized by postnatal muscle hypertrophy [145] resulting in a 30-40 % increase in protein production. Generation of Callipyge goats would help to: (1) answer a fundamental question if increase in protein production is directly linked to the mutation or require additional regulatory elements; and (2) potentially dramatically increase meat production in goats. Nuclear Transfer This subgroup of the Project (CT, IL, IN, LA, MD, MS, NC, UT) will focus on the procedures and underlying biology associated with significant advancements in the area of SCNT. Although scientists can successfully reprogram somatic cells prior to NT and produce live animals, there is a gap in knowledge regarding the inefficiency of the SCNT process (<10%), especially with respect to genetic modification. Aberrant patterns of DNA methylation, one of the major epigenetic modifications of the genome, have been proposed as a contributing factor in the poor development of embryos following SCNT. Certainly, epigenetic influences on progression of SCNT embryos will be further investigated as this area has moved to the forefront of science. Another concern with SCNT is the ability to maintain somatic cells in culture long enough for successful gene targeting and subsequent selection [134]. This group will evaluate culture conditions in an attempt to enhance the number of cloned embryos that develop to term, including addressing why somatic cell cultures often phase out by 25 to 28 passages. Next, the group realizes the importance of developing cells from livestock species to compare to cell lines in mice that develop into multiple tissue types. Finally, many developmental abnormalities are associated with SCNT procedures and systems for optimal survival post-transfer remain to be developed. This group will attempt to identify why gene expression patterns and morphology are abnormal in clones. One methodology will be to consider species differences. The developmental potential of hybrid NT embryos, such as sheep-cow, to produce viable offspring will be studied. Another approach is to evaluate the time NT embryo clones spend in vitro prior to being placed into an optimal in vivo environment. Would some abnormalities associated with bovine SCNT be overcome if cloned embryos were transferred earlier (i.e., surgically into the oviduct) rather than non-surgical transfer into the uterus after 5 to 6 days of culture? Another methodology will be to tag potentially important factors in somatic cells with fluorescent proteins and determine if these factors become reprogrammed following SCNT. UT group is working on identifying factors responsible for LOS phenotype after SCNT in cattle and sheep but not in goats by assessing gene expression and methylation status of imprinted genes. Gamete and Embryo Cryopreservation This subgroup of the Project (AR, CO, IA, LA, MT, NE) will develop methodologies for improvements in cryopreservation of sperm, oocytes and embryos, as well as gain a better understanding of the molecular and cellular processes influenced by freezing and thawing procedures. Investigators have recently focused on developing methods for cryopreservation of oocytes, which has presented many new challenges. Successful vitrification of porcine [131], equine and bovine oocytes [132] has been achieved, although the efficiency and success of these cryopreservation technologies remain low. The group will build on these successes to further enhance the efficiency of oocyte cryopreservation. Although the metaphase II spindle can be preserved during the slow freezing process, it is gradually disassembled during thawing of oocytes. Efforts will be concentrated on identifying cryoprotectants/proteins and cellular components that prevent disassembly of the metaphase II spindle during thawing. Oocytes will be fixed before, during and after cryopreservation and examined by immunocytochemistry and confocal microscopy. Further, this group will analyze differences in mammalian sperm cryopreservation. Male to male variation in the ability of sperm to survive the freezing and thawing process is significant and more investigation is required to identify the factors contributing to these differences in fertility. These researchers will cryopreserve sperm from males of different species/breeds with divergent fertility using established protocols and identify differences at the genomic, cellular and protein levels following the thawing process. Studies will not be limited to sperm obtained from standard collection methods but epididymal reservoirs of sperm will also be evaluated, including those of livestock animals that are injured or post-mortem. Finally, the group will continue to advance their knowledge base in regard to cryopreservation of embryos. Efficient production of genetically enhanced animals depends on successful protocols for short and long-term storage of manipulated embryos [56]. Consistent with this, cryopreservation of manipulated and/or IVF embryos need to be investigated and improved. The group will examine the effects of various manipulation methods (IVF, IVC, microinjection, assisted hatching) on the viability of embryos following freezing and thawing compared to standard in vivo-derived embryos. Novel tools for embryo manipulation and assisted cryopreservation are being developed by the group (MT). These are allowing successful cryopreservation of embryo “types” (i.e., either of different species or of different stages) that were previously refractory to this. These technologies will be further advanced here. Culture conditions will be critically examined, alternative cryoprotectants will be analyzed, molecular and cellular mechanisms will be compared and new protocols identified to make manipulated embryos more similar to in vivo-derived controls. In addition, new techniques for freezing smaller equine embryos (<300 ?m in diameter) by vitrification and slow cooling have been developed. The large equine embryo routinely collected non-surgically is particularly challenging to cryopreserve. This group will further evaluate and optimize these promising protocols. In addition, these techniques will be evaluated with embryos from other species. Another area of focus will be to determine why embryos from different genetic lines have divergent survival rates following vitrification and thawing. Following standard vitrification and thawing protocols, embryos from genetic lines with divergent survival rates will be examined to determine gene expression profiles, differences in proteins/factors associated with plasma membrane integrity, timing of detrimental effects following freezing/thawing, and whether alternative cell signaling/apoptotic pathways are activated/inhibited. Stem Cells This group of investigators (AR, CA, CO, CT, GA, IL, MD, NC, OR, UT, WA) will study biological mechanisms and procedures involved in isolation of both ES cells as well as tissue-specific stem cells. Our understanding of cellular differentiation has greatly expanded, largely due to the dramatic advances in SCNT methodologies. However, one major limitation to the livestock industry is the absence of ES cells, largely due to inappropriate culture conditions to maintain these undifferentiated cells appropriately. Isolation of ES cells in livestock species will continue to be of utmost importance to this scientific group. Procedures for isolation of mouse ES cells are well established and have been attempted with ES-like cells from livestock species. The group will focus on development of new, unique methods that might lead to an improvement in isolation of these cells in livestock animals as well as understanding the unique pathways of pluripotency maintenance that may be operating in livestock species. Finally, experiments by the CO workstation utilize trophoblast stem cells to gain further insight into the role of pluripotency factors in placental development and differentiation. Initial data obtained from studies in mice have now been used to advance our understanding of the genetic factors necessary for placenta formation in livestock animals. As an alternative to ES cells, much research expertise has been dedicated to the isolation of tissue-specific stem cells. This group has already isolated neural, amniotic, mesenchymal, and adipose-derived stem cells as well as pursued induced pluripotent stem (iPS) cells for livestock species. Member stations will collaborate on studies with mesenchymal and adipose-derived stem cells, focusing on epigenetic modification and trans-differentiation of these cells. Another station has developed a novel differentiation culture system that is capable of producing large numbers of germ-like cells that undergo advanced stages of development from ES cells. The group envisions major impacts of this system on the successful outcomes of NT as well as providing a source for renewal of gametes. Finally, this research collaboration will continue to explore the mechanisms underlying differentiation with particular attention to changes that occur upon formation of the ICM in blastocyst stage embryos. Members of the W3171 multistate project are working together, towards the common goals of improving the efficiency of natural livestock animal reproduction, as well as the production of transgenic livestock for food/fiber production and biomedical research purposes. No one person or laboratory or even station alone will provide all the answers, but rather many minds thinking and hands working towards the same goals while sharing successes and failures is likely to drive greater success. Table 1 (below) highlights and summarizes the multi-station participation in the various objectives and sub-objectives of the currently proposed W3171 research effort. Table 1. Collaboration summary by objective and sub-objective. Objective 1: Understand the biology and underlying mechanisms of gamete development, fertilization, and embryogenesis. Sub-objectives Stations involved Planned activities Oocyte Maturation and Developmental Competence CA, CT, CO, IL, LA, MS, NC, NE, NV, WV, UT Peer-reviewed scientific publications regarding: mechanisms of oocyte maturation, fertilization, and early embryonic development; and new approaches for IVM-IVF-IVD. Sperm Physiology AR, CA, CO, LA, MS, SC Fertilization Mechanisms CA, CO, IL, MS, NC, NV, UT, WV Early Embryonic Development AR, CA, CO, CT, IL, IN, MD, NC, NE, OR, WV, UT In Vitro Embryo Manipulation CA, CO, CT, IL, LA, NC, UT Objective 2: Refine methods for production of genetically enhanced animals to improve livestock production efficiency. Sub-objectives Stations involved Planned activities Transgenic Animals CT, IL, LA, MD, NE, UT Peer-reviewed scientific publications regarding: nuclear reprogramming and SCNT, gene editing and directed genomic modifications for improved livestock production, utility and methods for isolating pluripotent stem cells from domesticated species. Nuclear Transfer CT, IL, IN, LA, MD, MS, NC, UT Gamete and Embryo Cryopreservation AR, CO, IA, LA, MT, NE Stem Cells AR, CA, CO, CT, GA, IL, MD, NC, OR, UT, WA Over-arching plans include the training of future scientists in the art and biology of gamete and embryonic development. In addition, project members will present data to peer scientists and to other public venues. It is true that much – even most – of the research towards these end goals is performed at separate stations with the distinct (not shared) endpoints, outcomes, and impacts desired by that individual station. Yet the sponsored multi-state project format allows for substantial, regular interactions with potential collaborators – interactions that would not likely come about without the multi-state project. In the case of the W3171 group, these interactions have led to some very specific collaborative efforts that are a direct result of the multi-state research project. Just a few selected collaborations are mentioned in detail below: • Curt Youngs (IA) and Bob Godke (LA) – both long-time members of this multi-state project – have worked together for years on very applied aspects of animal reproductive technologies. Their efforts have resulted in six joint publications realized over the past 5 years. Their efforts have not only increased the efficiency of assisted reproductive technologies in livestock animals, but have increased the likelihood that these technologies will be adopted by every-day producers. • Carol Keefer (MD) has supplied plasmid DNA expression constructs essential to the production and/or characterization of induced pluripotent stem cells to Jianbo Yao (WV) and Cindy Tian (CT) and to researchers at AgResearch in New Zealand. Even this (seemingly) small collaborative effort has the potential to impact the research going forward within the multi-state group and around the world regarding the similarities and differences of induced pluripotent stem cells in livestock versus human and rodent model systems. • Brett White (NE) and Trish Berger (CA) are collaborating on an exciting new line of research to decipher the nature of the endocrine basis (if any) of the disruption of male fertility in swine that are deficient in GnRH Receptor II. • Matt Wheeler (IL) and Jim Murray (CA) are working together to generate and characterize novel lines of transgenic cattle and goats that will serve as models for human intestinal disease. These new animal models have the potential to pave the way for human pre-clinical and clinical research to save lives and/or improve the quality of life in humans with gastrointestinal dysfunction. • Clay Isom (UT) and Ken Bondioli (LA) are embarking on a nascent collaborative venture to utilize a technique for single-cell gene expression analysis available in Utah to test the expression level of genes involved in oocyte quality in individual oocytes from cattle housed in Louisiana and fed a rumen-protected conjugated linoleic acid. In summary, this proposal re-emphasizes two primary research areas (gamete physiology and early embryo development; and transgenic technologies), each with various sub-emphases. Each participating station has varying degrees of investment in the various research efforts, but all project participants are contributing – alone and collaboratively – in ways both large and small to the combined efforts of improving reproductive efficiency in domestic livestock species.Measurement of Progress and Results
Outputs
- Peer-reviewed scientific publications reporting novel contributions to the fields of gamete and embryo biology and generation of transgenic animals.
- • Generating novel information regarding the mechanisms of oocyte maturation, fertilization, and early embryo development.
- • New approaches for in vitro oocyte maturation, fertilization and culture of preimplantation embryos.
- • Improved understanding on the process of nuclear reprogramming during somatic cell nuclear transfer and normal developmental process.
- • New methodologies for genetic modifications of livestock species, with emphasis on gene editing and directed modifications.
- Output 6: Improved technologies for gamete and embryo cryopreservation. Output 7: Isolation and characterization of pluripotent stem cells from domesticated animal species. Output 8: Graduate and postdoctoral students trained on areas related to gamete and embryo biology and generation of transgenic animals. Output 9: Scientific pre-conference symposium where member of the groups will present their findings to their peers.
Outcomes or Projected Impacts
- Generate new scientific knowledge about the basic molecular, biochemical and cellular mechanisms of gamete and preimplantation embryo biology.
- Improve efficiency of in vitro embryo production system.
- Provide guidance for implementation of assisted reproductive technologies to commercial operations.
- Further the understanding of epigenetic reprogramming during early development, after somatic cell nuclear transfer, and during induction of pluripotency by defined factors.
- Generate germline-competent pluripotent stem cells from livestock species.
- The outcomes of this project can be grouped in three broad applications: 1) applied animal breeding to propagate animals with superior quantitative traits and/or pedigrees (“prime†grade steers, proven dairy bulls, disease resistance, e.g.); 2) a tool for basic research, (i.e., mechanisms of cellular differentiation and dedifferentiation), and 3) a biotechnological tool (more efficient approaches to produce genetically enhanced farm animals). Within these broader areas some of the projected outcomes include: Outcome/Impact 6: Develop and implement new approaches to introduce targeted genetic modifications to domesticated animal species. Outcome/Impact 7: Provide novel information to policymakers about the use and safety of transgenic animals for agricultural applications. Some specific examples of recent past, current, and anticipated outcomes and impacts from individual stations and station members affiliated with this project are as follows: Outcome/Impact 8:Advanced embryo manipulation technologies and tools being developed will be integrated into novel advanced teaching and training opportunities for graduate and undergraduate students. Outcome/Impact 9: These research findings will allow for more efficient semen freezing that should allow for more widespread implementation of artificial insemination and other reproductive technologies in horses, donkeys, and related species. Outcome/Impact 10: Research findings associated with heat stress in pigs will provide a very economical and practical way to improve reproductive performance during the heat of the summer months. Outcome/Impact 11: Assays to select high quality embryos will boost success rates and reduce costs associated with making cloned transgenic animals significantly. The economic impact of genetically enhanced animals to U.S. animal agriculture in the future cannot be estimated with any confidence. However, cash receipts for the livestock sector were projected to be totaling $183.4 billion during 2014 [146]. Quantitative genetics has led to impressive gains in livestock productivity, and assisted reproductive technologies have equivalent potential for improving livestock efficiency. Also, as animal production systems face new challenges, such us increasing high quality food demands for a growing human population, enhancing animal welfare, reducing environmental footprint, adjusting to changing climate, etc, it can only be expected that assisted reproductive technologies and targeted genetic modifications will be critical towards addressing these challenges.
Milestones
(2015): (2015) A collaborative research proposal for seeking funding from either a federal agency or philanthropic organization will be submitted.(2018): (2018) A half-day pre-conference symposium consisting of scientific presentations by W3171 members related to advances in gamete/embryo technologies will be presented to facilitate dissemination of project findings to the research community and ART practitioners. W3171 members who are also members of IETS will initiate discussion with IETS planning committee to organize the pre- symposium outreach activity. If this plan does not meet with IETS planning and organizing committee approval, then the W3171 members will work together to identify a suitable alternative to the planned pre-symposium outreach activity.
(2019): (2019) A review article related to each of the two objectives of this project will be published.
(0):ar the committee meets to share results from independent station and collaborative research projects. The research committee meets in conjunction with International Embryo Transfer Society (IETS) when the meeting is held in the USA, and elsewhere when the IETS holds its meeting outside of the USA.
Projected Participation
View Appendix E: ParticipationOutreach Plan
Project members of W3171 represent a broad geographical region with a varied and diversified agricultural base for each station. Regardless of environmental and production settings, understanding gamete and developmental biology of livestock will be a key factor in improving livestock efficiency. To that end, members are dedicated to communicating our discoveries and research findings to a host of constituency and peer groups. Students. Mentoring future scientists is one of our primary outreach methods. Undergraduate, graduate, and post-doctoral students conduct research in our laboratories and farms. During their training they practice scientific method, and hone their higher learning skills by analyzing, interpreting, writing, and presenting research findings. As an example of our commitment to student education, members of W3171 have led the education committee for International Embryo Transfer Society for more than two decades. In 2013, our members led IETS to develop an undergraduate student research competition at their 2014 international meeting. Over the years, many of the graduate student award winners at IETS presented projects that were part of W171, W1171, and W2171 the earlier versions of W3171. Public and Livestock Producers. Members of our project frequently present research findings and application to extension/teacher in-service education and training venues, and at livestock producer field days and symposia. Many of those events require either or both verbal and written interpretations of our research findings. For example, one of the primary outreach efforts conducted by the Iowa station involves publication of articles in the annual Iowa State University Animal Industry Report (available online at http://www.ans.iastate.edu/report/air/). This report is geared toward livestock producers, extension specialists, and members of the general public. The Iowa station has also published articles in various encyclopedias such as Encyclopedia of Biotechnology in Agriculture and Food and Encyclopedia of Animal Science that are geared toward a similar audience. The Iowa station also gives presentations pertaining to research conducted under this multi-state research project to livestock producer groups and community members. In similar fashion, the Illinois station sponsors Illini PorkNET (http://www.livestocktrail.illinois.edu/porknet/), the online resource for the Pork Industry, for many aspects of pork production, including reproduction and reproductive technologies. Technology transfer is an important service that we perform related to our individual productivity, but it is also a means of collaboration by inviting W3171 colleagues to speak at events not located at their home station. Extending our outreach to non-traditional groups includes presenting hands-on learning activities to students in junior high and high schools. The Maryland station has cooperated with a local school to bring laboratory exercises involving the characterization of embryonic stem cells to the high school science curriculum. The Nebraska station has performed over 30 workshops focused on Animal Biotechnology for junior and senior high school students, 4-H and FFA groups, and junior/community college students. The workshops describe methodologies for the production of transgenic livestock, emphasizing the power of such technologies to improve livestock animal production. These workshops are very popular and effective, assisting in the recruitment of many students to the Animal Science major at the University of Nebraska-Lincoln. Also, members of the Utah station pooled resources and effort to put on a university-sponsored hands-on community “science night” focused on assisted reproductive technologies in livestock animals, including cloning by somatic cell nuclear transfer and transgenesis. Utah station members are also major players in the Utah State University (USU) Center for Integrated BioSystems’ Annual Biotechnology Summer Academy for high school students, which is a structured program where current and recently-graduated high school students come to USU and participate in research and training in the life sciences. This year (2014) marks the thirteenth consecutive year of the summer academy, and will also be the first year for hosting a second academy session specifically targeted for students of Native American heritage from USU’s Blanding campus in the “Four-Corners” area of the American Southwest. Restricted budgets from federal and state sources require that we communicate our message and impact to politicians and constituents. Current and former members of W3171 have testified before the U.S. Congress on animal biotechnologies and hosted legislative delegations in our laboratories. The aforementioned examples of outreach will continue as part of our future plan. Scientific Community. Presenting at scientific meetings (regional, national, and international) and publishing research findings in peer-reviewed journals is a major component of our outreach plan. Members of W3171 hold membership and leadership roles in many professional organizations. Those organizations include: International Embryo Transfer Society, Society for the Study of Reproduction, American Society of Animal Science, American Dairy Science Association, and American Registry of Professional Animal Scientists; therefore, the impact of W3171 research is spread over many organizations. As stated in the milestones section, we will work with meeting organizers to develop symposia at professional meetings, primarily IETS, but others will be considered. Overall, W3171 members will use every opportunity to concisely and accurately explain how biological research and technologies can and will increase the efficiency of livestock production.
Organization/Governance
Governance of this Technical Committee will include the election of a Chair, a Vice-Chair, and a Secretary. Election of Secretary will occur annually. Secretary will rotate to Vice-Chair, and the Vice-Chair to Chair. The agenda for the annual meeting of the Technical Committee will be set by the Chair and he/she will preside over the meeting. The Secretary will prepare minutes of the annual meeting as well as the annual report. Administrative guidance will be provided by an assigned Administrative Advisor and a CSREES Representative.
Literature Cited
1. Vilsack TJ, et al. USDA Strategic Plan 2010-2015. In: Agriculture USDo (ed.). Washington, D.C., USA; 2009. 2. Beck M, Weigel JC, Englecke G, Patterson J, Randel L, Bryant T, Tablante T, Pettigrew J, Hess BW, Glenn B, Scarfe D. Farm Animal Integrated Research (FAIR) 2012. In: Societies FoAS (ed.). Champaign, Illinois; 2012. 3. Covey T. U.S. farm sector livestock cash receipts and value of production, 2009-2013F. In: Service U-ER (ed.). Washington, D.C., USA; 2012. 4. NASS. Statistics by State. In: Service U-NAS (ed.), vol. 2013. Washington, D.C., USA; 2012. 5. Wheeler MB. Production of transgenic livestock: promise fulfilled. Journal of Animal Science 2003; 81 Suppl 3:32-37. 6. Prather RS. Cloned transgenic heart-healthy pork? Transgenic Research 2006; 15:405-407. 7. Donovan DM, Kerr DE, Wall RJ. Engineering disease resistant cattle. Transgenic Research 2005; 14:563-567. 8. Tong J, Wei H, Liu X, Hu W, Bi M, Wang Y, Li Q, Li N. Production of recombinant human lysozyme in the milk of transgenic pigs. Transgenic Research 2011; 20:417-419. 9. Lyall J, Irvine RM, Sherman A, McKinley TJ, Nunez A, Purdie A, Outtrim L, Brown IH, Rolleston-Smith G, Sang H, Tiley L. Suppression of avian influenza transmission in genetically modified chickens. Science 2011; 331:223-226. 10. Forsberg EJ. Commercial applications of nuclear transfer cloning: three examples. Reproduction, Fertility, and Development 2005; 17:59-68. 11. Golovan SP, Meidinger RG, Ajakaiye A, Cottrill M, Wiederkehr MZ, Barney DJ, Plante C, Pollard JW, Fan MZ, Hayes MA, Laursen J, Hjorth JP, et al. Pigs expressing salivary phytase produce low-phosphorus manure. Nature Biotechnology 2001; 19:741-745. 12. Bähr A, Wolf E. Domestic Animal Models for Biomedical Research. Reproduction in Domestic Animals 2012; 47:59-71. 13. Whyte JJ, Prather RS. Genetic modifications of pigs for medicine and agriculture. Molecular Reproduction and Development 2011; 78:879-891. 14. Sullivan EJ, Pommer J, Robl JM. Commercialising genetically engineered animal biomedical products. Reproduction, Fertility, and Development 2008; 20:61-66. 15. Dai Y, Vaught TD, Boone J, Chen SH, Phelps CJ, Ball S, Monahan JA, Jobst PM, McCreath KJ, Lamborn AE, Cowell-Lucero JL, Wells KD, et al. Targeted disruption of the alpha1,3-galactosyltransferase gene in cloned pigs. Nature Biotechnology 2002; 20:251-255. 16. Lai L, Kolber-Simonds D, Park KW, Cheong HT, Greenstein JL, Im GS, Samuel M, Bonk A, Rieke A, Day BN, Murphy CN, Carter DB, et al. Production of alpha-1,3-galactosyltransferase knockout pigs by nuclear transfer cloning. Science 2002; 295:1089-1092. 17. Amiri Yekta A, Dalman A, Eftekhari-Yazdi P, Sanati MH, Shahverdi AH, Fakheri R, Vazirinasab H, Daneshzadeh MT, Vojgani M, Zomorodipour A, Fatemi N, Vahabi Z, et al. Production of transgenic goats expressing human coagulation factor IX in the mammary glands after nuclear transfer using transfected fetal fibroblast cells. Transgenic Research 2013; 22:131-142. 18. CAST. The role of transgenic livestock in the treatment of human disease. In: (CAST) CfASaT (ed.). Ames, Iowa; 2007. 19. Kuroiwa Y, Kasinathan P, Choi YJ, Naeem R, Tomizuka K, Sullivan EJ, Knott JG, Duteau A, Goldsby RA, Osborne BA, Ishida I, Robl JM. Cloned transchromosomic calves producing human immunoglobulin. Nature Biotechnology 2002; 20:889-894. 20. Kuroiwa Y, Kasinathan P, Matsushita H, Sathiyaselan J, Sullivan EJ, Kakitani M, Tomizuka K, Ishida I, Robl JM. Sequential targeting of the genes encoding immunoglobulin-mu and prion protein in cattle. Nature Genetics 2004; 36:775-780. 21. Thomson AJ, McWhir J. Biomedical and agricultural applications of animal transgenesis. Molecular Biotechnology 2004; 27:231-244. 22. Wheeler MB, Hurley WL, Mosley J, Bressner GE, Monaco E, Cake MM. 436 Risk analysis of ?-lactalbumin transgene transfer to nontransgenic control animals during rearing, breeding, parturition and lactation. Reproduction, Fertility and Development 2009; 22:375-375. 23. Tang MX, Zheng XM, Hou J, Qian LL, Jiang SW, Cui WT, Li K. Horizontal gene transfer does not occur between sFat-1 transgenic pigs and nontransgenic pigs. Theriogenology 2013; 79:667-672. 24. Farin PW, Slenning BD, Britt JH. Estimates of pregnancy outcomes based on selection of bovine embryos produced in vivo or in vitro. Theriogenology 1999; 52:659-670. 25. Papadopoulos S, Rizos D, Duffy P, Wade M, Quinn K, Boland MP, Lonergan P. Embryo survival and recipient pregnancy rates after transfer of fresh or vitrified, in vivo or in vitro produced ovine blastocysts. Animal Reproduction Science 2002; 74:35-44. 26. Pomar FJ, Teerds KJ, Kidson A, Colenbrander B, Tharasanit T, Aguilar B, Roelen BA. Differences in the incidence of apoptosis between in vivo and in vitro produced blastocysts of farm animal species: a comparative study. Theriogenology 2005; 63:2254-2268. 27. Rizos D, Clemente M, Bermejo-Alvarez P, de La Fuente J, Lonergan P, Gutierrez-Adan A. Consequences of in vitro culture conditions on embryo development and quality. Reproduction in Domestic Animals 2008; 43 Suppl 4:44-50. 28. Rizos D, Fair T, Papadopoulos S, Boland MP, Lonergan P. Developmental, qualitative, and ultrastructural differences between ovine and bovine embryos produced in vivo or in vitro. Molecular Reproduction and Development 2002; 62:320-327. 29. Blondin P, Bousquet D, Twagiramungu H, Barnes F, Sirard MA. Manipulation of follicular development to produce developmentally competent bovine oocytes. Biology of Reproduction 2002; 66:38-43. 30. Machaty Z, Day BN, Prather RS. Development of early porcine embryos in vitro and in vivo. Biology of Reproduction 1998; 59:451-455. 31. Peters JK, Milliken G, Davis DL. Development of porcine embryos in vitro: effects of culture medium and donor age. Journal of Animal Science 2001; 79:1578-1583. 32. Bauer BK, Isom SC, Spate LD, Whitworth KM, Spollen WG, Blake SM, Springer GK, Murphy CN, Prather RS. Transcriptional profiling by deep sequencing identifies differences in mRNA transcript abundance in in vivo-derived versus in vitro-cultured porcine blastocyst stage embryos. Biology of Reproduction 2010; 83:791-798. 33. Lonergan P, Rizos D, Gutierrez-Adan A, Fair T, Boland MP. Effect of culture environment on embryo quality and gene expression - experience from animal studies. Reprod Biomed Online 2003; 7:657-663. 34. Tesfaye D, Ponsuksili S, Wimmers K, Gilles M, Schellander K. A comparative expression analysis of gene transcripts in post-fertilization developmental stages of bovine embryos produced in vitro or in vivo. Reproduction in Domestic Animals 2004; 39:396-404. 35. Whitworth KM, Agca C, Kim JG, Patel RV, Springer GK, Bivens NJ, Forrester LJ, Mathialagan N, Green JA, Prather RS. Transcriptional profiling of pig embryogenesis by using a 15-K member unigene set specific for pig reproductive tissues and embryos. Biology of Reproduction 2005; 72:1437-1451. 36. Wrenzycki C, Herrmann D, Lucas-Hahn A, Korsawe K, Lemme E, Niemann H. Messenger RNA expression patterns in bovine embryos derived from in vitro procedures and their implications for development. Reproduction, Fertility, and Development 2005; 17:23-35. 37. Fauque P, Jouannet P, Lesaffre C, Ripoche MA, Dandolo L, Vaiman D, Jammes H. Assisted Reproductive Technology affects developmental kinetics, H19 Imprinting Control Region methylation and H19 gene expression in individual mouse embryos. BMC Developmental Biology 2007; 7:116. 38. Fernandez-Gonzalez R, Ramirez MA, Pericuesta E, Calle A, Gutierrez-Adan A. Histone modifications at the blastocyst Axin1(Fu) locus mark the heritability of in vitro culture-induced epigenetic alterations in mice. Biology of Reproduction 2010; 83:720-727. 39. Horii T, Yanagisawa E, Kimura M, Morita S, Hatada I. Epigenetic differences between embryonic stem cells generated from blastocysts developed in vitro and in vivo. Cell Reprogram 2010; 12:551-563. 40. Kafer GR, Kaye PL, Pantaleon M, Moser RJ, Lehnert SA. In vitro manipulation of Mammalian preimplantation embryos can alter transcript abundance of histone variants and associated factors. Cell Reprogram 2011; 13:391-401. 41. Maher ER. Imprinting and assisted reproductive technology. Human Molecular Genetics 2005; 14 Spec No 1:R133-138. 42. Rivera RM, Stein P, Weaver JR, Mager J, Schultz RM, Bartolomei MS. Manipulations of mouse embryos prior to implantation result in aberrant expression of imprinted genes on day 9.5 of development. Human Molecular Genetics 2008; 17:1-14. 43. Wright K, Brown L, Brown G, Casson P, Brown S. Microarray assessment of methylation in individual mouse blastocyst stage embryos shows that in vitro culture may have widespread genomic effects. Human Reproduction 2011; 26:2576-2585. 44. Mullins LJ, Wilmut I, Mullins JJ. Nuclear transfer in rodents. J Physiol 2004; 554:4-12. 45. Niemann H, Rath D, Wrenzycki C. Advances in biotechnology: new tools in future pig production for agriculture and biomedicine. Reproduction in Domestic Animals 2003; 38:82-89. 46. Wang B, Zhou J. Specific genetic modifications of domestic animals by gene targeting and animal cloning. Reprod Biol Endocrinol 2003; 1:103. 47. Keefer CL. Lessons learned from nuclear transfer (cloning). Theriogenology 2008; 69:48-54. 48. Oback B. Climbing Mount Efficiency--small steps, not giant leaps towards higher cloning success in farm animals. Reproduction in Domestic Animals 2008; 43 Suppl 2:407-416. 49. Oback B, Wells DN. Donor cell differentiation, reprogramming, and cloning efficiency: elusive or illusive correlation? Molecular Reproduction and Development 2007; 74:646-654. 50. Bonk AJ, Cheong HT, Li R, Lai L, Hao Y, Liu Z, Samuel M, Fergason EA, Whitworth KM, Murphy CN, Antoniou E, Prather RS. Correlation of developmental differences of nuclear transfer embryos cells to the methylation profiles of nuclear transfer donor cells in swine. Epigenetics 2007; 2:179-186. 51. Isom SC, Whitworth K, Bonk A, Fergason EA, Prather RS. A comparison of global DNA methylation patterns in developmentally-competent vs -incompetent nuclear transfer donor cells. In: Society for the Study of Reproduction - Annual Meeting, vol. 77. San Antonio, TX: Biology of Reproduction; 2007. 52. Bosch P, Pratt SL, Stice SL. Isolation, characterization, gene modification, and nuclear reprogramming of porcine mesenchymal stem cells. Biology of Reproduction 2006; 74:46-57. 53. Gomez MC, Pope CE, Kutner RH, Ricks DM, Lyons LA, Ruhe MT, Dumas C, Lyons J, Dresser BL, Reiser J. Generation of domestic transgenic cloned kittens using lentivirus vectors. Cloning Stem Cells 2009; 11:167-176. 54. Monzani PS, Sangalli JR, De Bem TH, Bressan FF, Fantinato-Neto P, Pimentel JR, Birgel-Junior EH, Fontes AM, Covas DT, Meirelles FV. Breeding of transgenic cattle for human coagulation factor IX by a combination of lentiviral system and cloning. Genet Mol Res 2013; 12:3675-3688. 55. Gaj T, Gersbach CA, Barbas CF, 3rd. ZFN, TALEN, and CRISPR/Cas-based methods for genome engineering. Trends in Biotechnology 2013; 31:397-405. 56. Dinnyes A, Nedambale TL. Cryopreservation of manipulated embryos: tackling the double jeopardy. Reproduction Fertility and Development 2009; 21:45-59. 57. USDA/NIFA. Animal Reproduction Overview. In: Animals & Animal Products, vol. 2014. Washington, DC: National Institute of Food and Agriculture; 2012. 58. Inchaisri C, Jorritsma R, Vos PL, van der Weijden GC, Hogeveen H. Economic consequences of reproductive performance in dairy cattle. Theriogenology 2010; 74:835-846. 59. Nations U. World Population Prospects: The 2012 Revision. In: Department of Economic and Social Affairs PD (ed.). New York City, NY: United Nations; 2013. 60. Shaw PW, Bernard AG, Fuller BJ, Hunter JH, Shaw RW. Vitrification of mouse oocytes using short cryoprotectant exposure: effects of varying exposure times on survival. Molecular Reproduction and Development 1992; 33:210-214. 61. Wood MJ, Barros C, Candy CJ, Carroll J, Melendez J, Whittingham DG. High rates of survival and fertilization of mouse and hamster oocytes after vitrification in dimethylsulphoxide. Biology of Reproduction 1993; 49:489-495. 62. Zhu SE, Kasai M, Otoge H, Sakurai T, Machida T. Cryopreservation of expanded mouse blastocysts by vitrification in ethylene glycol-based solutions. Journal of Reproduction and Fertility 1993; 98:139-145. 63. Fulka J, Jr., First NL, Moor RM. Nuclear and cytoplasmic determinants involved in the regulation of mammalian oocyte maturation. Mol Hum Reprod 1998; 4:41-49. 64. Adashi EYLPCK. The Ovary. New York: Raven Press; 1993. 65. Conti M, Andersen CB, Richard F, Mehats C, Chun SY, Horner K, Jin C, Tsafriri A. Role of cyclic nucleotide signaling in oocyte maturation. Mol Cell Endocrinol 2002; 187:153-159. 66. Rose RD, Gilchrist RB, Kelly JM, Thompson JG, Sutton-McDowall ML. Regulation of sheep oocyte maturation using cAMP modulators. Theriogenology 2013; 79:142-148. 67. Mehlmann LM. Stops and starts in mammalian oocytes: recent advances in understanding the regulation of meiotic arrest and oocyte maturation. Reproduction 2005; 130:791-799. 68. Mehlmann LM, Saeki Y, Tanaka S, Brennan TJ, Evsikov AV, Pendola FL, Knowles BB, Eppig JJ, Jaffe LA. The Gs-linked receptor GPR3 maintains meiotic arrest in mammalian oocytes. Science 2004; 306:1947-1950. 69. Moon JH, Hyun CS, Lee SW, Son WY, Yoon SH, Lim JH. Visualization of the metaphase II meiotic spindle in living human oocytes using the Polscope enables the prediction of embryonic developmental competence after ICSI. Hum Reprod 2003; 18:817-820. 70. Rienzi L, Ubaldi F, Martinez F, Iacobelli M, Minasi MG, Ferrero S, Tesarik J, Greco E. Relationship between meiotic spindle location with regard to the polar body position and oocyte developmental potential after ICSI. Hum Reprod 2003; 18:1289-1293. 71. Krisher RL. The effect of oocyte quality on development. J Anim Sci 2004; 82 E-Suppl:E14-23. 72. Ferreira EM, Vireque AA, Adona PR, Meirelles FV, Ferriani RA, Navarro PA. Cytoplasmic maturation of bovine oocytes: structural and biochemical modifications and acquisition of developmental competence. Theriogenology 2009; 71:836-848. 73. Coticchio G, Sereni E, Serrao L, Mazzone S, Iadarola I, Borini A. What criteria for the definition of oocyte quality? Ann N Y Acad Sci 2004; 1034:132-144. 74. Combelles CM, Racowsky C. Assessment and optimization of oocyte quality during assisted reproductive technology treatment. Semin Reprod Med 2005; 23:277-284. 75. Wang Q, Sun QY. Evaluation of oocyte quality: morphological, cellular and molecular predictors. Reprod Fertil Dev 2007; 19:1-12. 76. Au HK, Yeh TS, Kao SH, Tzeng CR, Hsieh RH. Abnormal mitochondrial structure in human unfertilized oocytes and arrested embryos. Ann N Y Acad Sci 2005; 1042:177-185. 77. Brevini TA, Vassena R, Francisci C, Gandolfi F. Role of adenosine triphosphate, active mitochondria, and microtubules in the acquisition of developmental competence of parthenogenetically activated pig oocytes. Biol Reprod 2005; 72:1218-1223. 78. Hsieh RH, Tsai NM, Au HK, Chang SJ, Wei YH, Tzeng CR. Multiple rearrangements of mitochondrial DNA in unfertilized human oocytes. Fertil Steril 2002; 77:1012-1017. 79. Gibson TC, Kubisch HM, Brenner CA. Mitochondrial DNA deletions in rhesus macaque oocytes and embryos. Mol Hum Reprod 2005; 11:785-789. 80. Alm H, Torner H, Lohrke B, Viergutz T, Ghoneim IM, Kanitz W. Bovine blastocyst development rate in vitro is influenced by selection of oocytes by brillant cresyl blue staining before IVM as indicator for glucose-6-phosphate dehydrogenase activity. Theriogenology 2005; 63:2194-2205. 81. Sutovsky P. Sperm-egg adhesion and fusion in mammals. Expert Rev Mol Med 2009; 11:e11. 82. Wassarman PM. Mammalian fertilization: the strange case of sperm protein 56. Bioessays 2009; 31:153-158. 83. Parrish JJ. Bovine in vitro fertilization: in vitro oocyte maturation and sperm capacitation with heparin. Theriogenology 2014; 81:67-73. 84. Peddinti D, Nanduri B, Kaya A, Feugang JM, Burgess SC, Memili E. Comprehensive proteomic analysis of bovine spermatozoa of varying fertility rates and identification of biomarkers associated with fertility. BMC Syst Biol 2008; 2:19. 85. Etkovitz N, Tirosh Y, Chazan R, Jaldety Y, Daniel L, Rubinstein S, Breitbart H. Bovine sperm acrosome reaction induced by G-protein-coupled receptor agonists is mediated by epidermal growth factor receptor transactivation. Dev Biol 2009; 334:447-457. 86. Pate BJ, White KL, Winger QA, Rickords LF, Aston KI, Sessons BR, Li GP, Campbell KD, Weimer B, Bunch TD. Specific integrin subunits in bovine oocytes, including novel sequences for alpha 6 and beta 3 subunits. Mol Reprod Dev 2007; 74:600-607. 87. Misirlioglu M, Page GP, Sagirkaya H, Kaya A, Parrish JJ, First NL, Memili E. Dynamics of global transcriptome in bovine matured oocytes and preimplantation embryos. Proc Natl Acad Sci U S A 2006; 103:18905-18910. 88. Kues WA, Carnwath JW, Niemann H. From fibroblasts and stem cells: implications for cell therapies and somatic cloning. Reprod Fertil Dev 2005; 17:125-134. 89. Davis DL. Culture and storage of pig embryos. J Reprod Fertil Suppl 1985; 33:115-124. 90. Hansen PJ, Block J. Towards an embryocentric world: the current and potential uses of embryo technologies in dairy production. Reprod Fertil Dev 2004; 16:1-14. 91. Lonergan P, Fair T. The ART of studying early embryo development: progress and challenges in ruminant embryo culture. Theriogenology 2014; 81:49-55. 92. Wirtu G, Pope CE, Damiani P, Miller F, Dresser BL, Short CR, Godke RA, Bavister BD. Development of in-vitro-derived bovine embryos in protein-free media: effects of amino acids, glucose, pyruvate, lactate, phosphate and osmotic pressure. Reprod Fertil Dev 2003; 15:439-449. 93. Rizos D, Gutierrez-Adan A, Perez-Garnelo S, De La Fuente J, Boland MP, Lonergan P. Bovine embryo culture in the presence or absence of serum: implications for blastocyst development, cryotolerance, and messenger RNA expression. Biol Reprod 2003; 68:236-243. 94. Vanroose G, Van Soom A, de Kruif A. From co-culture to defined medium: state of the art and practical considerations. Reprod Domest Anim 2001; 36:25-28. 95. Abe H, Hoshi H. Evaluation of bovine embryos produced in high performance serum-free media. J Reprod Dev 2003; 49:193-202. 96. Harvey AJ. The role of oxygen in ruminant preimplantation embryo development and metabolism. Anim Reprod Sci 2007; 98:113-128. 97. Palasz AT, Beltran Brena P, De la Fuente J, Gutierrez-Adan A. The effect of bovine embryo culture without proteins supplements until day 4 on transcription level of hyaluronan synthases, receptors and mtDNA content. Zygote 2010; 18:121-129. 98. Krisher RL, Wheeler MB. Towards the use of microfluidics for individual embryo culture. Reprod Fertil Dev 2010; 22:32-39. 99. Jenuwein T, Allis CD. Translating the histone code. Science 2001; 293:1074-1080. 100. Brown R, Strathdee G. Epigenomics and epigenetic therapy of cancer. Trends Mol Med 2002; 8:S43-48. 101. Bogliotti YS, Ross PJ. Mechanisms of histone H3 lysine 27 trimethylation remodeling during early mammalian development. Epigenetics 2012; 7:976-981. 102. Rivera RM, Ross JW. Epigenetics in fertilization and preimplantation embryo development. Prog Biophys Mol Biol 2013; 113:423-432. 103. Dean W, Santos F, Stojkovic M, Zakhartchenko V, Walter J, Wolf E, Reik W. Conservation of methylation reprogramming in mammalian development: aberrant reprogramming in cloned embryos. Proc Natl Acad Sci U S A 2001; 98:13734-13738. 104. Shi W, Haaf T. Aberrant methylation patterns at the two-cell stage as an indicator of early developmental failure. Mol Reprod Dev 2002; 63:329-334. 105. Rodriguez-Osorio N, Wang Z, Kasinathan P, Page GP, Robl JM, Memili E. Transcriptional reprogramming of gene expression in bovine somatic cell chromatin transfer embryos. BMC Genomics 2009; 10:190. 106. Hill JR, Winger QA, Long CR, Looney CR, Thompson JA, Westhusin ME. Development rates of male bovine nuclear transfer embryos derived from adult and fetal cells. Biol Reprod 2000; 62:1135-1140. 107. Hill JR, Burghardt RC, Jones K, Long CR, Looney CR, Shin T, Spencer TE, Thompson JA, Winger QA, Westhusin ME. Evidence for placental abnormality as the major cause of mortality in first-trimester somatic cell cloned bovine fetuses. Biol Reprod 2000; 63:1787-1794. 108. Cibelli J. Developmental biology. A decade of cloning mystique. Science 2007; 316:990-992. 109. Han YM, Kang YK, Koo DB, Lee KK. Nuclear reprogramming of cloned embryos produced in vitro. Theriogenology 2003; 59:33-44. 110. Beyhan Z, Forsberg EJ, Eilertsen KJ, Kent-First M, First NL. Gene expression in bovine nuclear transfer embryos in relation to donor cell efficiency in producing live offspring. Mol Reprod Dev 2007; 74:18-27. 111. Beyhan Z, Ross PJ, Iager AE, Kocabas AM, Cunniff K, Rosa GJ, Cibelli JB. Transcriptional reprogramming of somatic cell nuclei during preimplantation development of cloned bovine embryos. Dev Biol 2007; 305:637-649. 112. Smith SL, Everts RE, Tian XC, Du F, Sung LY, Rodriguez-Zas SL, Jeong BS, Renard JP, Lewin HA, Yang X. Global gene expression profiles reveal significant nuclear reprogramming by the blastocyst stage after cloning. Proc Natl Acad Sci U S A 2005; 102:17582-17587. 113. Wilmut I, Sullivan G, Taylor J. A decade of progress since the birth of Dolly. Reprod Fertil Dev 2009; 21:95-100. 114. Kuroiwa Y, Kasinathan P, Sathiyaseelan T, Jiao JA, Matsushita H, Sathiyaseelan J, Wu H, Mellquist J, Hammitt M, Koster J, Kamoda S, Tachibana K, et al. Antigen-specific human polyclonal antibodies from hyperimmunized cattle. Nat Biotechnol 2009; 27:173-181. 115. Men H, Walters EM, Nagashima H, Prather RS. Emerging applications of sperm, embryo and somatic cell cryopreservation in maintenance, relocation and rederivation of swine genetics. Theriogenology 2012; 78:1720-1729. 116. Amann RP. Treatment of sperm to predetermine sex. Theriogenology 1989; 31:49-61. 117. Johnson LA, Cran DG, Polge C. Recent advances in sex preselection of cattle: Flow cytometric sorting of X- & Y-chromosome bearing sperm based on dna to produce progeny. Theriogenology 1994; 41:51-56. 118. de Graaf SP, Evans G, Maxwell WM, Cran DG, O'Brien JK. Birth of offspring of pre-determined sex after artificial insemination of frozen-thawed, sex-sorted and re-frozen-thawed ram spermatozoa. Theriogenology 2007; 67:391-398. 119. Curran S. Fetal Sex Determination in Cattle and Horses by Ultrasonography. Theriogenology 1992; 37:17-21. 120. da Cruz AS, Silva DC, Costa EO, De MJP, da Silva CC, Silva DM, da Cruz AD. Cattle fetal sex determination by polymerase chain reaction using DNA isolated from maternal plasma. Anim Reprod Sci 2012; 131:49-53. 121. Yang H, Zhong FG, Yang YL, Wang XH, Liu SR, Zhu B. Sex determination of bovine preimplantation embryos by oligonucleotide microarray. Animal Reproduction Science 2013; 139:18-24. 122. Chitwood JL, Rincon G, Kaiser GG, Medrano JF, Ross PJ. RNA-seq analysis of single bovine blastocysts. BMC Genomics 2013; 14. 123. Berthelot F, Martinat-Botte F, Locatelli A, Perreau C, Terqui M. Piglets born after vitrification of embryos using the open pulled straw method. Cryobiology 2000; 41:116-124. 124. Carnevale EM. Vitrification of equine embryos. Veterinary Clinics of North America-Equine Practice 2006; 22:831-+. 125. Hong QH, Tian SJ, Zhu SE, Feng JZ, Yan CL, Zhao XM, Liu GS, Zheng SM. Vitrification of boer goat morulae and early blastocysts by straw and open-pulled straw method. Reproduction in Domestic Animals 2007; 42:34-38. 126. Seidel GE. Modifying oocytes and embryos to improve their cryopreservation. Theriogenology 2006; 65:228-235. 127. Somfai T, Ozawa M, Noguchi J, Kaneko H, Nakai M, Maedomari N, Ito J, Kashiwazaki N, Nagai T, Kikuchi K. Live Piglets Derived from In Vitro-Produced Zygotes Vitrified at the Pronuclear Stage. Biology of Reproduction 2009; 80:42-49. 128. Oberstein N, O'Donovan MK, Bruemmer JE, Seidel GE, Carnevale EM, Squires EL. Cryopreservation of equine embryos by open pulled straw, cryoloop, or conventional slow cooling methods. Theriogenology 2001; 55:607-613. 129. Dattena M, Ptak G, Loi P, Cappai P. Survival and viability of vitrified in vitro and in vivo produced ovine blastocysts. Theriogenology 2000; 53:1511-1519. 130. Massip A. Cryopreservation of embryos of farm animals. Reproduction in Domestic Animals 2001; 36:49-55. 131. Liu Y, Du YT, Lin L, Li J, Kragh PM, Kuwayama M, Bolund L, Yang HM, Vajta G. Comparison of efficiency of open pulled straw (OPS) and Cryotop vitrification for cryopreservation of in vitro matured pig oocytes. Cryoletters 2008; 29:315-320. 132. Hurtt AE, Landim-Alvarenga F, Seidel GE, Squires EL. Vitrification of immature and mature equine and bovine oocytes in an ethylene glycol, Ficoll and sucrose solution using open-pulled straws. Theriogenology 2000; 54:119-128. 133. Blomberg LA, Telugu BP. Twenty years of embryonic stem cell research in farm animals. Reprod Domest Anim 2012; 47 Suppl 4:80-85. 134. Denning C, Priddle H. New frontiers in gene targeting and cloning: success, application and challenges in domestic animals and human embryonic stem cells. Reproduction 2003; 126:1-11. 135. Prelle K, Zink N, Wolf E. Pluripotent stem cells--model of embryonic development, tool for gene targeting, and basis of cell therapy. Anat Histol Embryol 2002; 31:169-186. 136. Wheeler MB, Malusky SA. Transgenesis in mammals: status and perspectives. Acta Scientiae Veterinariae 2003; 31:119-136. 137. Gjorret JO, Maddox-Hyttel P. Attempts towards derivation and establishment of bovine embryonic stem cell-like cultures. Reprod Fertil Dev 2005; 17:113-124. 138. Wells DN, Oback B, Laible G. Cloning livestock: a return to embryonic cells. Trends Biotechnol 2003; 21:428-432. 139. Trounson A. Derivation characteristics and perspectives for mammalian pluripotential stem cells. Reprod Fertil Dev 2005; 17:135-141. 140. Oback B. Cloning from stem cells: different lineages, different species, same story. Reprod Fertil Dev 2009; 21:83-94. 141. West FD, Machacek DW, Boyd NL, Pandiyan K, Robbins KR, Stice SL. Enrichment and differentiation of human germ-like cells mediated by feeder cells and basic fibroblast growth factor signaling. Stem Cells 2008; 26:2768-2776. 142. Telugu BP, Ezashi T, Roberts RM. Porcine induced pluripotent stem cells analogous to naive and primed embryonic stem cells of the mouse. Int J Dev Biol 2010; 54:1703-1711. 143. Christian M, Cermak T, Doyle EL, Schmidt C, Zhang F, Hummel A, Bogdanove AJ, Voytas DF. Targeting DNA double-strand breaks with TAL effector nucleases. Genetics 2010; 186:757-761. 144. Miller JC, Tan S, Qiao G, Barlow KA, Wang J, Xia DF, Meng X, Paschon DE, Leung E, Hinkley SJ, Dulay GP, Hua KL, et al. A TALE nuclease architecture for efficient genome editing. Nat Biotechnol 2011; 29:143-148. 145. Cockett NE, Jackson SP, Shay TL, Farnir F, Berghmans S, Snowder GD, Nielsen DM, Georges M. Polar overdominance at the Ovine callipyge locus. Science 1996; 273:236-238. 146. Schnepf R. US Farm Income. In: Congressional Research Service (ed.). Washington, DC; 2014.