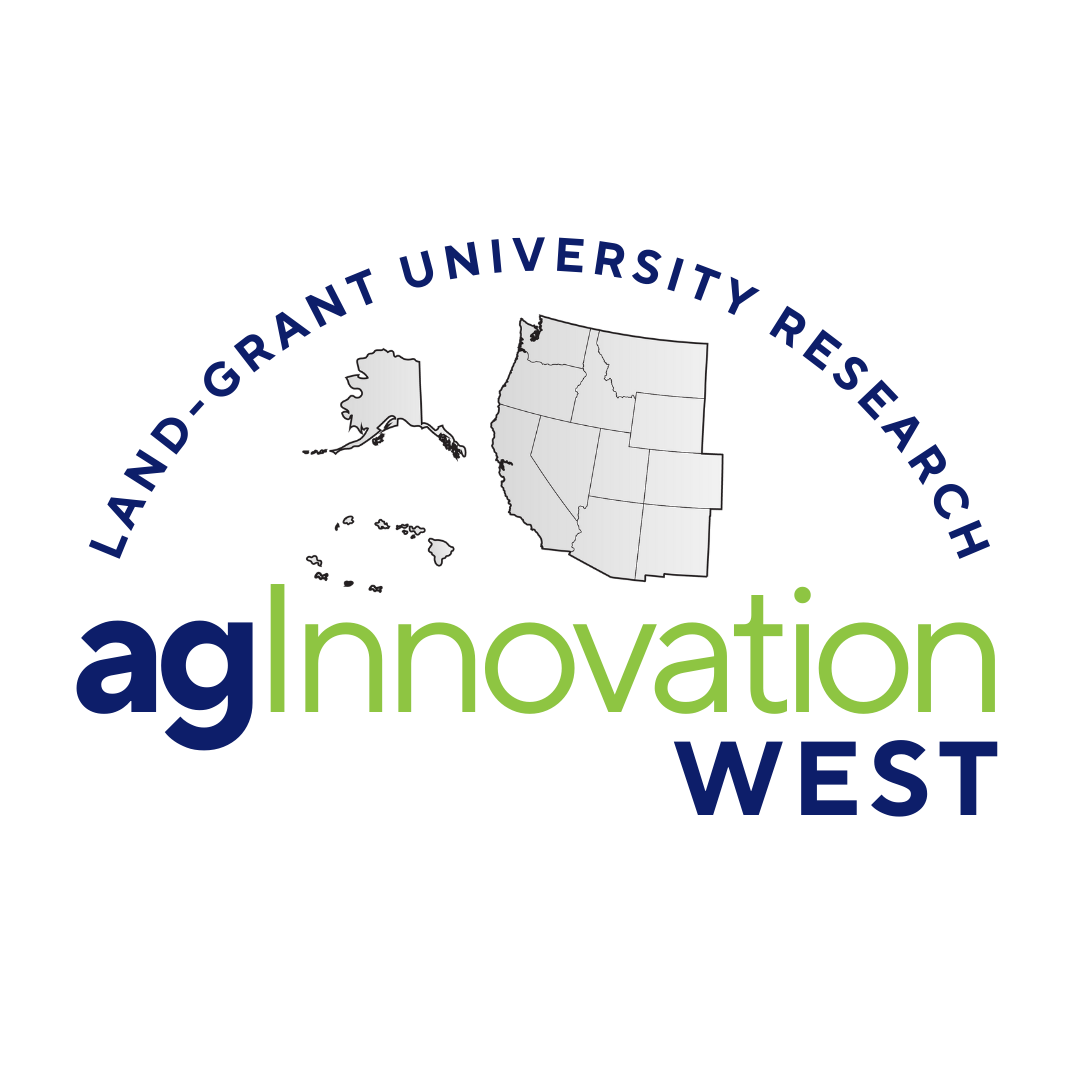
W2010: Integrated Approach to Enhance Efficiency of Feed Utilization in Beef Production Systems
(Multistate Research Project)
Status: Inactive/Terminating
W2010: Integrated Approach to Enhance Efficiency of Feed Utilization in Beef Production Systems
Duration: 10/01/2013 to 09/30/2018
Administrative Advisor(s):
NIFA Reps:
Non-Technical Summary
Statement of Issues and Justification
The overall goal of this cooperative, multi-state, multidisciplinary, research and outreach project is to improve beef cattle feed efficiency (FE) through more complete understanding of the underlying molecular mechanisms that contribute to its variation. The research scope of this project will include studies of the molecular and systems physiology, genetics/genomics, nutritional and behavioral interactions that drive variation in FE measured in terms of both Gain:Feed (G:F) and residual feed intake (RFI) . Through a strong integrated outreach program this regional project will coordinate efforts across the nation to provide leadership and interact with industry to cooperatively disseminate this important information to US beef cattle producers. This proposal for renewal of the W-1010 project describes the collaborative effort of researchers from Agricultural Experiment Stations across the nation to characterize specific aspects of the molecular physiological and genomic mechanisms that regulate beef cattle feed efficiency. Major points that support the continuation of this important, fundamental research project for the next five years are: 1. The project relates to a major agricultural problem. Meeting consumer needs for a high quality product while maintaining profitability of production, decreasing environmental impacts, and minimizing use of natural resources will require improvements in the efficiency of meat production in beef cattle. 2. The project relates directly to the objectives of the NIFA Strategic Plan 2010-2015: Objective 1.1 Enhance Rural Prosperity Objective 1.3 Support a Sustainable and Competitive Agricultural System Objective 3.1 Ensure U.S. Agricultural Resources Contribute to Enhanced Global Food Security Objective 3.2 Enhance Americas Ability to Develop and Trade Agricultural Products derived from new Technologies. These objectives are directly addressed in the following ways: In an era of unprecedented costs of feedstuffs, the importance of finding new ways to maintain production levels with reduced demand for feed will have a direct effect on rural prosperity and contribute to the sustainability of beef production. Reducing the demand for feedstuffs for similar production will also facilitate the distribution of feedstuffs for broader utilization. These technologies are readily transferable to beef production systems throughout the world and thus have potential to contribute to global food security. Realized reduction of the cost of production also translates to lower cost of U.S beef production resulting in more competitively priced beef products in the global marketplace. 3. National importance of building this multi-state project: Across the nation, individual research and outreach efforts with FE at the focal point, are rapidly expanding. Industry at multiple levels is embracing FE as a priority for development. In addition, the synergy that will be developed across this large team of researchers will convert projects with local or regional impact to having national and international impact. 4. All projects outlined in the following Methods section are technically sound and are in different stages of progress. Many of these have been peer reviewed and are presently contributing impact at the state, regional or local levels. 5. This project is both a multi-state and a multidisciplinary project, involving the effort of investigators with broad expertise across the nation and international collaborators in Australia and Canada. The Principal Investigators represent a variety of science disciplines and outreach expertise that complement each other and provide the skill-sets necessary to complete the objectives. 6. The project will involve strong cooperative efforts between the various units including exchange of reagents, cDNA probes, and an FE phenotype database as well as the sharing of knowledge and techniques, joint use of equipment and techniques available at particular stations, and joint publication of research results. Numerous collaborative projects are described in the procedures for the proposal. The committee feels strongly that the collaborations in this project would have been substantially more difficult to establish and maintain outside the framework of a funded regional project. 7. The members of the committee have been highly successful in obtaining outside support to fund the research. Funding from the NIFA, and other granting agencies, and industry sources has been essential to carry out the work and to maintain the high level of productivity of the group, and this record of outside funding is expected to continue. 8. This project describes a research and outreach approach to an important agricultural problem. The investigators at the cooperating stations have already demonstrated a high level of productivity, and are, thus, capable of making substantial progress towards the objectives outlined in this project proposal. 9. The impacts from completion of this multi-state project will be national and international in scope. This project will contribute scientific rigor in elucidating the molecular mechanisms that underpin variation in beef cattle feed efficiency and contribute to development of biologically-based predictors and improve the accuracy and reliability of mathematic models to predict feed intake in beef cattle. Producers will benefit from development of molecular technologies that enhance conventional EPD estimates. The developed technologies will value-add to conventional phenotyping for feed efficiency which is costly and time-consuming. Thus the technologies are expected to lead to longer-term reduction in costs of overall FE testing and improve the FE of beef cattle resulting in lower impact on the environment, and reducing costs to the producer for similar levels of beef production. 10. The W-1010 Committee continues to be highly productive. A total of 71 papers, book chapters/theses and abstracts have resulted from years 2008-2011 of the current five-year project, and additional publications are expected by the September 30, 2013, termination date. In addition, substantial progress has been made under each of the specific objectives of the current project.
Related, Current and Previous Work
Mechanisms by which animals vary in their ability to partition dietary energy into maintenance and productive functions are described in the ensuing paragraphs. Animals that are better able to digest feeds and extract nutrients should be more efficient at converting feed into product(37). Animals that consume more feed tend to be more efficient (in terms of G:F or feed conversion ratio, FCR), due to the dilution of maintenance(3). Conversely, RFI methodology takes maintenance into account, so that differences in RFI are more likely to be due to variations in maintenance energy expenditures or basal energy metabolism per se. The components of basal metabolism include organism-level service functions, such as respiratory, circulatory and excretory work; macromolecule turnover; and ion transport. Since many of these processes occur at intensities that vary widely among tissues, the proportions of metabolically active organs (i.e., viscera such as liver, GI tract, kidneys, etc.) relative to less active tissues (e.g., adipose) could also affect energy partitioning. The energy required to deposit a unit of lipid is actually quite similar to that required to deposit the same amount of protein, because although lipid has a higher energy density, protein has a lower net efficiency of deposition and those differences tend to cancel each other out. However, once deposited, maintenance energy required for adipose tissue is low while for active muscle, the energy demand is much higher(1, 31). Animals that are less efficient in terms of RFI, tend to be fatter(57, 61). Macromolecule turnover is considered a component of maintenance. Growing animals accumulate protein, lipid and carbohydrate because the rates of synthesis of these components exceed the rates of degradation(32). Based on stoichiometry of relevant metabolic pathways, it appears that protein turnover is a major contributor to energy expenditures, with carbohydrate turnover playing a much smaller role and lipid turnover having a negligible impact on energy utilization. There are genetic differences in the rates of these processes and in the activities of the associated enzymes. Moreover, all of these processes are subject to endocrine, paracrine and autocrine hormonal regulation. The role of the rumen and gastrointestinal tract microbes are critical to the ruminants performance as these microbes provide the animal with its nutritional needs in the form of volatile fatty acids and microbial cell protein (37, 44). There is an imperative need to study the microbial populations that resides within ruminants to understand what roles microbes play to mediate the balance between health and disease and to find new ways to increase animal performance. Animals actually break down molecules from feed and capture the energy obtained for use in other functions in the form of high energy phosphate bonds (e.g. ATP). Theoretically, the electron transport pathway should generate three high-energy phosphate bonds for each NADH or NADPH molecule and two high-energy phosphate bonds for each FADH2 delivered to the mitochondrial membrane. In reality, the proton gradient across the membrane is insufficient to achieve those yields, and actual yields are closer to 2.5 a 1.5. The leak of protons along the gradient is thought to be responsible for this(11, 39). Clearly, variations in the yield of high energy phosphate bonds that are required to fuel metabolic and productive functions will result in variations in feed efficiency. Differences in RFI are partially due to variations in tissue-level maintenance energy expenditures(58), cell-level extent of protein turnover(58), and capacity to generate optimal proton gradients across mitochondrial membranes to maximize efficiency of ATP production(9, 11). Each of these processes is dependent on the complementary presence and function of a requisite set of enzymatic and transport proteins for maximal metabolic efficiency. Therefore, differences in the expression of proteins responsible for coordinating the regulated expression of canonical pathways, synthesis of cell and organelle components and/or environments in which these proteins function, and that regulate gene expression and/or the function of gene expression products, may way well exist between high and low FE animals. Several pathways have been shown to be directly associated with variation in metabolic function, as a direct response to genomic variation (SNPs). It appears possible that polymorphisms in the leptin gene may be associated with animal performance (FCR and RFI). Single nucleotide polymorphisms have been reported in the bovine leptin gene(13, 19). Others(25) have also reported positive associations between microsatellite marker BM1500 and body fatness in cattle. The leptin gene SNP is more highly represented in British breeds versus continental breeds(53). The feed efficiency literature provides insight into relative energetic efficiency of fat versus muscle. We use the example of RFI, as it has been shown by several studies that less efficient animals (in terms of RFI) tend to be fatter while more RFI-efficient animals tend to be leaner(14, 52). In fact, some authors who study RFI also include a correction for body fat in the model that predicts feed intake(2, 4, 65), as it is widely understood that at least 5% of the variation in RFI is due to differences in body composition(58). There is also evidence to suggest that relative muscle mass is increased more efficient animals. Basarab et al.(6) found a modest correlation with dissectible carcass lean content (r = -0.17). Great variation in composition of skeletal muscle tissue exists both within an individual muscle tissue and among all muscle tissues of the mammalian body. Muscle function and size are important factors involved in the variation of composition; however, muscle fiber type is considered a major contributor to variation. Studies of the role of fiber type are needed to further understand the contribution of muscle tissue to variation in feed efficiency. Maintenance of skeletal muscle metabolism requires considerable energy on the part of the animal, depending on fiber type and composition of muscle mass(17). Skeletal muscle accounts for approximately 40-45% of the total body mass of vertebrates, regardless of their body size(7). When considering the whole animal, skeletal muscle can contribute approximately 60% or more to systemic metabolism. Thus, skeletal muscle is one of the most energy-consuming tissues. The mammalian body has the ability to selectively shuttle and oxidize substrates across different tissues based on necessity and uptake efficiency of the tissue. There are three distinct and identifiable mechanisms that regulate this substrate partitioning. First, substrate mobilization includes processes in adipose tissue, liver, and muscle, resulting in reversal of the energy storage processes gluconeogenesis, lipolysis, and glycogenolysis. Second, substrate uptake includes groups of mechanisms by which substrates enter each cell type. Third, substrate oxidation is a highly regulated process that determines which substrate will be utilized and their relative rates of utilization. Except heavy or prolonged exercise, the bodys capacity for substrate uptake exceeds oxidation rate. Oxidation rate reflects demand of tissues. Therefore, regulation of oxidation rate is the critical factor of all three of the aforementioned mechanisms. It appears that some actions of the hormones leptin and insulin may be antagonistic as insulin inhibits oxidation of free fatty acids and leptin appears to suppress this insulin effect(12, 16, 48, 49). Evidence for the intersection of the leptin and insulin pathways is also supported as an increase in fatty acid oxidation in muscle (associated with insulin resistance); appears to be linked to an increase in diacylglycerol synthesis and activation of protein kinase C(8, 27, 73); a reduction in insulin-stimulated IRS-1-associated PI3-kinase activity, a blunting of insulin-stimulated IRS-1 tyrosine phosphorylation(27), and inhibition of glucose transport and glucose phosphorylation(63). The effects of leptin treatment on muscle may be partially attenuated by a synthetic blockade of PI3-kinase activity(49). Leptin also directly stimulates fatty-acid oxidation in muscle by activating the 5-AMP-activated protein kinase (AMPK), an enzyme that phosphorylates and subsequently inactivates acetyl CoA carboxylase(46). Solinas et al. (66) propose that leptin may stimulate thermogenesis in skeletal muscle via a mechanism independent of decoupling of beta oxidation from ATP synthesis (through UCPs). In this scenario, leptin may induce a futile cycling between de novo lipogenesis and lipid oxidation, a process also requiring glucose and thus interdependence on insulin signaling. It is proposed that AMPK in response to leptin and PI3-kinase in response to insulin play pivotal roles in this process. Thus, this appears to be a potential mechanism that underlies individual variation in energetic (and feed) efficiency. Metabolic regulation plays a critical role in the underlying variation of feed efficiency. Richardson and Herd(59) determined that energy expenditures such as tissue metabolism accounted for 37% of the variation in RFI and defined another 27% of the variation as other. Residual feed intake has been identified as a polygenic trait, which only intensifies the complexity of this feed efficiency concept. However, recent scientific investigations (10, 15, 60) are beginning to indicate possible metabolic mechanisms that may be associated with feed efficiency. Skeletal muscle is a unique tissue in that it has adaptive capabilities which allow it to alter composition and function in response to different physiological conditions. There are two main adaptations or changes that will be discussed in this section: (1) metabolic plasticity and (2) muscle fiber plasticity (anabolic growth mainly regulated via the insulin-like growth factor-1 (IGF-I) axis(21)). Each of these changes can dramatically affect processes occurring within skeletal muscle and, as a result, alter the function of the muscle. More importantly, due to the role of skeletal muscle in terms of feed efficiency, it is critical that we understand how each of these conditions affect not only skeletal muscle at the tissue level, but also how it contributes to the overall growth of an animal. In addition, understanding how it might affect energetic (and feed) inputs required to grow the muscle or sustain it in its remodeled form is equally as important. Mitochondrial biogenesis is one of the most important alterations concerning metabolic plasticity. Mitochondrial biogenesis consists of two types of inclusive alterations within the muscle cell: there is an increase in mitochondrial content per gram of tissue, and/or, there is a change in the mitochondrial composition(11, 34). These alterations are highly specific and occur in response to particular types of exercise (i.e., resistance vs. endurance), with changes exhibited most evidently in low-oxidative, white muscle (type IIb) fibers(35). In order to initiate mitochondria biogenesis in skeletal muscle, a series of signaling events must occur, which include elevation of intracellular calcium (Ca2+) and activation of Ca2+-sensitive signaling molecules, activation of gene transcription that encodes mitochondrial proteins, messenger ribonucleic acid (mRNA) translation into protein, and protein structure assembly(33). The consequences of mitochondrial biogenesis are metabolically beneficial for skeletal muscle at the cellular level. Since skeletal muscle is such an energy-demanding tissue, an increase in the number of mitochondria will allow for cellular metabolic preference to utilize high-energy lipid substrates instead of carbohydrates (i.e., glucose and glycogen). Ultimately, this partitioning will sustain glycogen stores within muscle, reduce the formation of lactic acid (due to an increase in aerobic capabilities), and reduce muscle fatigue(34). Skeletal muscles with a high capacity for lipid oxidation will ultimately exhibit greater efficiency for the mobilization of adipose (fat) storage and have an increased endurance rate. One of the most important topics to discuss is the endocrinology of growth(21, 71). An important regulator of skeletal muscle growth is the somatotropic axis, which consists of growth hormone (GH), IGF-I and IGF-II, binding proteins (IGF binding proteins 1-6 and GH binding protein), and receptors. Each factor exhibits a precise role within the axis to influence the growth of skeletal muscle; however, the production and regulation of each factor is an integrated process within the mammalian endocrine system. Insulin-like growth factor-I plays a critical role in both prenatal and postnatal growth; additionally, IGF-II is also a major activator of prenatal growth, but its role in postnatal growth is yet to be understood. Therefore, the following discussion will focus on the growth-promoting effects of IGF-I at the local (muscle tissue) level. Scientific investigations of the past (64) revealed that the liver is the primary source of circulating IGF-I (endocrine/systemic production), however a reduction in circulating IGF-I does not necessarily inhibit postnatal growth(72). This discovery led to speculation that IGF-I production was occurring elsewhere within the body, meaning that growth was not completely dependent upon hepatic IGF-I production. In fact, IGF-I is produced both systemically and at the tissue level, and it is now known that both endocrine and autocrine/paracrine effects of IGF-I are important for growth(42, 50). Local IGF-I production occurs via autocrine and paracrine effects. In the context of skeletal muscle tissue, IGF-I production by an individual muscle cell can act upon itself to continue the cellular release of IGF-I or act upon other cells within a close proximity to produce IGF-I. Further, IGF binding proteins (IGFBPs) are also produced locally within skeletal muscle tissue. Skeletal muscle expresses IGFBPs 2, 4, 5, and 6(26). The major functions of these binding proteins are to assist in the transport of IGFs both locally and systemically, to prolong the half-life of IGFs and regulate plasma clearance, and to modulate the interactions of IGFs with their receptors. The binding proteins of IGFs can either potentiate or inhibit the actions of IGFs; however, IGFBP-4 is thought to be exclusively inhibitory(38). Both in vivo and in vitro studies have demonstrated that local and systemic production of IGF-I is involved in postnatal myofiber hypertrophy and muscle regeneration. In skeletal muscle, over-expression of the IGF-I gene promotes local muscle hypertrophy and can also delay atrophy of muscle fibers associated with ageing(55). In both instances, IGF-I improves muscle mass and strength. Furthermore, skeletal muscle has the ability to regenerate after damage, due to skeletal muscle satellite cells(22). Satellite cells have been defined as adult stem cells residing in a tissue-specific area, such as skeletal muscle(40). Due to the effects of IGF-I on skeletal muscle hypertrophy, IGF-mediated actions on satellite cells regarding muscle regeneration are being actively investigated (56). It has been suggested that induced IGF-I skeletal muscle hypertrophy and regeneration is due to local production of IGF-I via autocrine and/or paracrine effects rather than circulating IGF-I(5). Furthermore, IGF-I has been implicated as a stimulator of protein synthesis and an inhibitor of protein degradation, thus improving protein retention(54). Indicators of growth and feed efficiency have become important topics of the beef industry, especially during trying times of high feed prices and elevated demand for product. The ability to measure IGF-I serum levels on a large number of cattle before making important management decisions makes it a desirable genetic indicator. It has been documented that serum IGF-I has an estimated heritability of 32% and is positively correlated with important production traits(20). Further research is needed to determine if the IGF-I axis will become well understood and provide reliable indicators for improved feed efficiency of beef cattle. The thyroid gland produced thyroxine and tri-iodothyronine which reduce muscle efficiency and increases metabolism. Tri-iodothyronine increases energy expenditure(41). Increased feed intake in cattle is related to greater plasma concentration of thyroxine(18). Alterations in control of thyroid secretions nd function may occur in animals that require greater energy intake to maintain body weight. The improvement of lean to fat deposition ratio can lower feed costs for producers by increasing feed efficiency of animals(53). However, product quality and palatability are of considerable importance to the consumer, therefore beef producers have focused on providing high cost (high energy) diets with the aim of maintaining or improving product quality and to date have borne the cost (dollars and energy cost) of providing high energy diets. Each depot of adipose tissue differs in its metabolic regulation. Trayhurn and Beattie(68) reported in human preadipocytes isolated from different fat depots, the omental depot has a higher lipid flux than the subcutaneous depot. The rate of triglyceride mobilization is also greater within visceral as opposed to omental fat depots. Furthermore, omental preadipocytes have a higher glucocorticoid receptor density, lipoprotein lipase activity, adenylyl cyclase activity, and apoptotic properties than subcutaneous adipose tissue. Subcutaneous adipocytes have been shown to have higher levels of GLUT 4, glycogen synthase, and insulin receptors, as well as a higher rate of differentiation when compared to omental adipocytes(51). These authors also reported that omental cells expressed higher levels of Cellular Inhibitor of Apoptosis Protein (cIAP), an anti-apoptotic protein, than subcutaneous adipocytes. This suggests that cells from different depots show inherent differences that account for the observed physiological variation between adipocytes isolated from different locations(51). There are metabolic differences between intramuscular and subcutaneous fat depots(45). Others(23, 43) also reported that in cattle, intramuscular adipocytes are smaller than subcutaneous fat cells. Additionally, activities of the glycolytic enzymes hexokinase and phosphofructokinase were higher in intramuscular depots. Subcutaneous fat depots had higher levels of the lipogenic enzymes NADP-malate dehydrogenase, 6-phosphogluconate dehydrogenase, and glucose 6-phosphate dehydrogenase. This indicates that different adipose depots have unique roles in and contributions to overall lipid metabolism. Breed also appears to be a factor in the lipogenic activity of the tissue depot(43, 45). Intermuscular fat depots have similar characteristics to intramuscular fat depots. Eguinoa et al.(23) reported that in cattle, the intermuscular fat depot had the smallest adipocyte size when compared to omental, peri-renal, and subcutaneous depots. The intermuscular depot additionally had a lower level of lipogenic enzyme activity than other depots, as was the case in intramuscular fat depots. However, when adjusting for adipocyte size, subcutaneous and intermuscular fat depots had higher enzyme activity than the other depots. This indicates a potential role of other factors such as blood flow and lipolytic activity as determinants of depot differences observed. Vasculature development precedes adipose tissue growth and adipose tissue has the ability to grow throughout the lifetime of the animal, therefore Hutley et al.(36) hypothesized that microvascular endothelial cells may secrete location specific factors that regulate adipose tissue growth. These factors may play a role in depot differences seen in adipose tissue. Endothelial cells stimulated preadipocyte proliferation, but there were negligible differences in differentiation between different depots(36). Researchers have compared adipogenic factors from cells isolated from subcutaneous abdominal, omental, and mesenteric fat depots(47, 69). Subcutaneous preadipocytes developed the most, mesenteric cells were intermediate, and omental cells had the lowest levels of lipid, GDPH activity, and adipocyte fatty acid binding protein (aP2). Levels of the transcription factors PPAR-³ and C/EBP-± followed the same order of expression. There appears to be higher leptin mRNA levels in subcutaneous than omental fat depots, but this may be due to adipocyte volume, as it has been reported that leptin is correlated to adipocyte volume, and subcutaneous adipocytes are larger than omental adipocytes(74). This variation in fat depot metabolism and adipocyte size and physiological dynamics indicates that as animals vary in the relative proportions of fat that are accumulated across different depots, the energetic dynamics of their overall metabolism will vary. Thus, variation in fat depots is a potential difference at tissue level that can contribute to variation in maintenance requirements and feed efficiency. Variation in adipocyte growth exists throughout animal development. During normal growth of beef cattle, omental fat reaches its maximal growth rate first, followed by intermuscular and then subcutaneous fat. Intramuscular fat depots grow during the later maturation stage of animals and are responsible for the characteristic marbling found in beef carcasses. Intramuscular fat is highly valued by producers and the wider industry as it provides an excellent indicator of meat palatability. Thus, high intramuscular fat levels indicate a high quality product. As noted above, there is a considerable energetic price associated with producing a high quality product with high intramuscular fat levels. Evidence in the feed efficiency literature suggests that it is very important to consider the potential antagonism in selecting for RFI that may lead to inadvertent selection for leaner animals. However further review of literature provides details of studies in which selection for low post-weaning RFI animals did not compromise product quality (24, 28, 29, 62, 67, 70). This discussion illustrates the great importance of marbling and thus the need for ongoing monitoring and to further study of the relationship between FE and marbling. ________________________________________ A search for overlap with other multistate activities revealed a few projects with distinctly different objectives to the present proposal: NC1181 Sustaining Forage-based Beef Cattle Production in a Bioenergy Environment; NC1182 Nitrogen Cycling, Loading, and Use Efficiency in Forage-Based Livestock Production Systems; NC1184 Molecular Mechanisms Regulating Skeletal Muscle Growth and Differentiation; NCCC210 Regulation of Adipose Tissue Accretion in Meat-Producing Animals; NRSP009 National Animal Nutrition Program; S1045 Genetic Considerations for Beef Cattle Production in Challenging Environments; SERA041 Beef Cattle Production Utilizing Forages in the Southeast to Integrate Research and Extension Programs across State Boundaries; W1012 Improving ruminant use of forages in sustainable production systems for the western U.S.; and WERA001 Beef Cattle Breeding in the Western Region. However, many of the objectives of these other projects are complimentary to those of the present multi-state project proposal. Whenever appropriate, committee members will maintain communication with these groups through collaborative research efforts and attendance at meetings of these groups.
Objectives
-
To understand biological sources of variation in efficiency of feed utilization.
-
To discover physiological biomarkers and genetic markers for feed efficieny.
-
To develop EPDs, informed by molecular studies in building multi-trait selection indices and decision-support tools to facilitate selection for improved feed efficiency in beef cattle
-
To develop producer educational programs to enhance technology adoption by the beef industry.
Methods
By working cooperatively across this multi-state project, scientists in the participating Experiment Stations will build synergy and will expand their focus from the regional and state levels to have national and international impact. 1. To understand biological sources of variation in efficiency of feed utilization. Studies under this objective will focus primarily on identification of the sources and quantification of the magnitude of the biological drivers of variation in FE. The following Experiment Stations will work in collaboration on this objective: Alabama, Arizona, California, Colorado, Florida, Idaho, Illinois, Kentucky, Missouri, Nebraska, Tennessee and Texas. Previous research has shown that differences in RFI are related to variations in multiple mechanisms including variations in ruminal fermentation patterns and digestibility, as well as variation in maintenance energy requirement, which in turn is related to rates of body protein turnover. Collaboratively, we will determine the range in RFI that exists within the beef population. Outlier animals will be used to identify hormonal, cellular respiration, digestion and energetic differences responsible for differences in RFI among animals. Protein will be extracted from liver and skeletal muscle tissue to compare the effect of RFI extreme phenotype on the relative content of critical gatekeeper proteins of targeted metabolic pathways. Likewise, RNA will be extracted from these outlier animals and targeted and genomic gene expression profiles compared to determine the effect of RFI phenotype on known and elucidated regulatory and canonical gene networks. DNA will also be collected from these animals to be used in objective 2. These experiment stations will also produce matings from sires phenotyped for RFI. The offspring from these matings will be used to determine the impact of selection for RFI on efficiency of cattle managed on forage and concentrate diets and performance of feedlot cattle and females retained as cows as described under objective 3. Research with yearling steers from known parental RFI matings has demonstrated differences in forage intake and utilization between negative and positive RFI sired bulls. Potential intake and metabolic efficiency driven differences in gain when forage was lush and plentiful as well as when forage was more mature and limiting were evident. Other preliminary research demonstrated that diet formulations can reduce manure volume output by 60% during the growth phase and 40% during the fattening phase of feedlot production. Research has also shown that intake within a population of beef cattle varies by 1.4 fold. Because waste output is a function of feed intake, the potential to select for efficient animals that are 40% more efficient would be expected to reduce waste output and methane emission by the same margin. The Florida, Idaho, Illinois, Missouri and Texas Experiment Stations will collaborate with New South Wales (Australia) to measure the difference in waste volume and methane output in animals that are efficient (negative RFI), average (RFI ~ 0), and inefficient (positive RFI). These data will be used to determine the potential improvement that can be made in waste output reductions via selecting for RFI. These determinations will be made for forage-based diets and grain-based diets designed to maximize efficiency. These measurements will also be made on offspring generated from matings using negative and positive RFI sires. These data will be used to determine the potential improvement that can be made from genetic selection for improved efficiency (negative RFI). The geographical diversity of the participating experiment stations will allow us to RFI phenotype progeny from the same bulls that are raised in widely different environments. DNA will also be collected from progeny born from sub-tropical to cool temperate environments. This will provide information on the effect of environment in estimating RFI. 2. To discover physiological biomarkers and genetic markers for RFI. Establishment of well-characterized populations of beef cattle across member experiment station herds and collaborating industry herds will provide the resource for this objective. In addition, outcomes from Objective 1, understanding the biological drivers of variation in RFI will contribute knowledge of candidate mechanistic pathways and candidate genes for study of potential physiological biomarkers and gene markers for RFI. The Alabama, Colorado, Idaho, Missouri, North Carolina, Ohio, Texas, West Virginia and Wyoming Experiment Stations will collaborate to identify genetic and physiological biomarkers for ranking RFI phenotypes of cattle. The Idaho, Colorado Missouri, Ohio and Texas Experiment Stations will lead the study of blood metabolites and hormonal profiles unique to RFI phenotype. As referenced above previous research has demonstrated that IGF-I from blood taken at weaning in Bos taurus cattle is correlated with RFI phenotype. A standard commercially available ELISA will be used to assay plasma IGF-I. The Ohio Station began divergently selecting for blood serum IGF-I concentration in purebred Angus cattle in 1989. Beginning with the 2009 breeding season, the selection criterion in the IGF-I selection lines was changed from serum IGF-I concentration to maintenance energy (ME) EPD as provided by the Red Angus Association. Females in the high line are mated to one of three high (undesirable) ME EPD bulls and cows and heifers in the low line are mated to one of three low (desirable) ME EPD bulls. The first calves produced in this project at the Ohio Station were born in the spring 2010 calving season. These divergent selection lines will be characterized for differences in RFI via collaboration with the Texas Experiment Station. Missouri and Texas will lead research studying the relationship of mitochondrial respiration with RFI phenotype. Previous research demonstrated that rate of oxygen uptake by the mitochondria was correlated with RFI phenotype. Therefore attempts will be made to develop a high-throughput assay to measure oxygen uptake or to identify respiratory chain proteins differences that can be used as markers for RFI phenotype. The Oklahoma Experiment Station will determine maintenance energy requirements of mature cows by individual feeding and adjusting diets to maintain body weight during late gestation. Endocrine (IGF-I and thyroxine) and metabolic biomarkers will be established for the most and least efficient cows and tissue samples will be available to determine genetic markers. In closely related work, the North Carolina Experiment Station will characterize bulls that have been phenotyped for RFI by studying plasma thyroxine and tri-iodothyronine. In preliminary studies, these hormonal markers have been found to be highly correlated with dry matter intake. In addition the roles of these markers in bulls studied at North Carolina, will also be studied in bulls phenotyped for RFI in Idaho, Ohio, and Texas. Furthermore, the Alabama and Idaho Experiment Stations will collaborate in understanding environmental interactions with these biological drivers. This collaboration will provide cattle raised in cool temperate and subtropical environments to investigate potential environmental influences on these key biological drivers. 3. To develop EPDs, informed by molecular studies in building multi-trait selection indices and decision-support tools to facilitate selection for improved FE in beef cattle. Studies under this objective will integrate molecular-level information in developing quantitative tools for the evaluation of FE and its implementation into industry. A major objective of this project will be the integration of data from populations of beef cattle in the development of high accuracy EPDs for RFI and other production traits. Advanced concepts in mathematical modeling of RFI will also be studied under this objective. Specifically, the California and Texas Experiment Stations will evaluate and re-parameterize existing mechanistic nutrition models to explain more of the individual variation in nutrient requirements and intake to facilitate selection programs to identify animals that have divergent differences in efficiency of feed utilization. Data from experiments conducted by participating Experiment Stations will be used to test and improve existing simulation models of cattle growth and body composition. Models will be modified by identifying and quantifying model parameters that reflect efficiency differences between animals, allowing estimation of system effects of selection or feeding animals of different efficiencies. Modeling analyses will also serve to evaluate hypotheses and extend interpretation of results regarding RFI, and also as a tool for extending knowledge regarding RFI to the beef industry and the public at large. As the multi-state project database incorporating phenotypic and genotypic information is developed, new iterations of performance models will be developed to interface with this database. This will enable improvement of the existing models as well as development of decision-aid tools to maximize application of those data by industry. Economic analysis predicting the profitability of adoption of feed efficiency in selections programs will be conducted by the Idaho and Utah Experiment Stations. 4. To develop producer educational programs to enhance technology adoption by the beef industry. Faculty participating in this multi-state project have research, teaching and extension appointments. Outreach will be a strong thrust of our multi-state efforts. Participation of members in a BIF National Standards sub-committee for development of feed efficiency testing protocols and interaction with state cattle associations and national breed societies will contribute to the integrative character of the project. In addition, participants will develop workshops and short courses designed to inform and aid producers in integrating selection for improved feed efficiency into current breeding programs and selection practices. North Carolina Experiment Station will lead the development of a workshop that will evolve from a series of presentations to industry participants and scientists. These presentations and workshop will be modeled after the Applied Reproductive Strategies in Beef Cattle workshops offered by the Applied Reproduction Taskforce. This multi-state project will facilitate broader interaction of extension faculty bringing national impact to our outreach efforts involving: Alabama, California, Idaho, Mississippi, Missouri and Texas Experiment Stations.Measurement of Progress and Results
Outputs
- Publications will be the primary tangible result expected from the project.
- Extensions publications derived from the primary scientific publications and other sources such as the project database will provide an important link that will lead to increased industry adoption nation-wide, and improved FE in beef cattle.
- The Committee will sponsor a Symposium on Molecular Mechanisms underlying FE. This symposium will provide a mechanism to convey new research information and will increase the visibility of the project.
- Interaction of the committee with the seed-stock industry will enable development of multi-breed EPDs for FE.
- Increased integration and mechanisms for sharing of information between the scientific community engaged in the study of FE and the beef industry sectors.
Outcomes or Projected Impacts
- Increased understanding of the role of growth factors and cell signaling pathways in regulating metabolic processes may lead to molecular- and cellular biology-based strategies to aid in selection of more feed-efficient beef cattle which will benefit both producers and consumers.
- Elucidation of specific genetic variation including SNPs responsible for variation in feed efficiency may provide information necessary to utilize genetic selection or molecular- or cell biology-based methods to manipulate metabolic pathways in growing beef cattle. For example, it may be possible to reduce protein degradation in growing muscle, which appears to be one of the mechanisms underpinning variation in FE. This will allow more rapid muscle growth without increasing energy requirements.
- Gene expression studies may also lead to identification of genes that may be used to produce transgenic animals that grow more efficiently. Additionally, because this project combines the skills of physiologists, nutritionists, muscle biologists, molecular biologists, geneticists, and outreach specialists, collaboration amongst committee members should result in integration of knowledge from the whole animal level to the level of the gene and/or cell in the elucidation of mechanisms that contribute to whole animal variation in feed efficiency.
- Research supported by this project will result in training of new researchers equipped to integrate FE research with molecular physiology and molecular genomics methods to study specific pathways and regulatory factors and the genes that contribute to variation in FE.
- Increased knowledge of the role of specific molecular physiological mechanisms, genes and gene variants in regulating feed efficiency in beef cattle.
Milestones
(2017): Sponsor a symposium on mechanisms underlying variation in RFI at an ASAS/ADSA National Meeting. This will allow us to convey research findings to interested individuals in these organizations.(2018): Develop a joint funding proposal including all participants who wish to contribute.
Projected Participation
View Appendix E: ParticipationOutreach Plan
Project participants have a history of information sharing activities, cooperation, and productivity. Many of the project scientists have been involved in similar areas previously (e.g. WCC-92, W-1010), some for decades. Their expertise and productivity are well documented by previous publications. Several of the scientists involved in this project have Extension appointments. This will enhance the dissemination of research results to the general public through presentations and popular publications. There are no artificial boundaries where the research component waits for the outreach function to do something with the information generated from project efforts. It is the intention of the technical committee that new questions and information should flow freely between the laboratory and industry to benefit the ultimate consumer. Most project scientists interact with outreach personnel at their respective institutions and at regional and national levels. Many of the aforementioned "Outcomes or Projected Impacts" lend themselves to outreach education activities. Various media can be used to educate producers, processors, policy makers, and consumers. It is intended that preparation of peer reviewed publications, presentations at professional meetings, and other means of disseminating project results to professional audiences be part of the ongoing project effort. This group also will work collaboratively with industry and government leaders to provide additional insight into solutions for many of the emerging challenges facing the livestock and meat industries. This will be accomplished by inviting these individuals to annual meetings and requesting their input into research directions for the future and their critique of ongoing research. When appropriate, published proceedings, or other published work will be released to the appropriate audiences. Further, we will extend our findings about RFI via models that will be integrated in producer software and outreach activities, such as beef performance projection and ration formulation computer programs. Thus the economics of use of animals with different RFI can be quantified. It is anticipated that utilization of these published results will lead to improved efficiency of lean meat production in domestic livestock and poultry. Additionally, in 2017 the committee will organize a symposium on molecular mechanisms that underlie variation in FE for the joint annual meeting of ASAS and ADSA that will convey recent research findings to the members of these organizations.
Organization/Governance
The Technical Committee will consist of at least one representative from each participating unit, appointed as described in the Manual for Cooperative Regional Research, with one representative from each unit designated as the voting member. The Western Regional Association of Directors will appoint one Director to serve as Administrative Advisor and a representative of the USDA/NIFA will also serve as an Advisor; both will be ex officio member of this committee. The Technical Committee will elect a Chair and a Secretary to serve for a period of one year, and these officers will continue to be voting members. In succeeding years, the Secretary will become Chair, and only a new Secretary will be elected. The Chair, the Secretary, the immediate Past Chair and the Administrative Advisor will serve as the Executive Committee, and they will have the authority to act on behalf of the Technical Committee during the periods between meetings. The Chair, with approval of the Administrative Advisor, will call yearly meetings of the Technical Committee and interim meetings of the Executive Committee if needed. A report of research results from each unit will be presented orally and in writing at the Technical Committee meetings. The written report should include a list of publications resulting from research related to the project for the year. Reports will be critically reviewed by the Technical Committee and recommendations will be made for future research and coordination of research between units to maximize attainment of the Objectives. The Secretary will prepare minutes and an Annual Report for distribution to Technical Committee members, Directors and Department Chairs of participating State Agricultural Experiment Stations and USDA Laboratories, and the Regional Research Office, NIFA. Because submission of a yearly progress report is essential to assess progress, ensure participation, and prepare the Annual Report, any unit that does not submit a written progress report for two consecutive years will be considered not to be participating and will be dropped from the project.
Literature Cited
1. Ahola JK, Campbell LT, Szasz JI, Skow TA, Hunt CW, Glaze JB, Jr., and Hill RA. Relationship between residual feed intake and meat quality in steer progeny of divergent intramuscular fat EPD Angus bulls. Journal of Animal Science 85: 167-168, 2007. 2. Ahola JK, Skow TA, Hunt CW, and Hill RA. Relationship between residual feed intake and end product palatability in longissimus steaks from steers sired by Angus bulls divergent for intramuscular fat expected progeny difference. Professional Animal Scientist 27: 109-115, 2011. 3. Arthur PF, Archer JA, Herd RM, Richardson EC, Exton SC, Wright JH, Dibley KCP, and Burton DA. Genetic and phenotypic variation in feed intake, feed efficiency and growth in beef cattle. In: Association for the Advancement of Animal Breeding and Genetics Proceedings of the Twelfth Conference. Dubbo, NSW: 1997, p. 234-237. 4. Baker SD, Szasz JI, Klein TA, Kuber PS, Hunt CW, Glaze JB, Jr., Falk D, Richard R, Miller JC, Battaglia RA, and Hill RA. Residual feed intake of purebred Angus steers: effects on meat quality and palatability. J Anim Sci 84: 938-945, 2006. 5. Bamman MM, Shipp JR, Jiang J, Gower BA, Hunter GR, Goodman A, McLafferty CL, and Urban RJ. Mechanical load increases muscle IGF-I and androgen receptor mRNA concentrations in humans. American Journal of Physiology - Endocrinology And Metabolism 280: E383-E390, 2001. 6. Basarab JA, Price MA, Aalhus JL, Okine EK, Snelling WM, and Lyle KL. Residual feed intake and body composition in young growing cattle. Canadian Journal of Animal Science 83: 189-204, 2003. 7. Blaxter K. Muscular Work. In: Energy Metabolism in Animals and Man Cambridge, UK: University Press, 1989, p. 147-179. 8. Boden G, Lebed B, Schatz M, Homko C, and Lemieux S. Effects of acute changes of plasma free fatty acids on intramyocellular fat content and insulin resistance in healthy subjects. Diabetes 50: 1612-1617, 2001. 9. Bottje WG, and Carstens GE. Association of mitochondrial function and feed efficiency in poultry and livestock species. In: Journal of Animal Science2008. 10. Bottje WG, and Carstens GE. Association of mitochondrial function and feed efficiency in poultry and livestock species. J Anim Sci 87: E48-63, 2009. 11. Bottje WG, and Carstens GE. Variation in Metabolism: Biological Efficiency of Energy Production and Utilization That Affects Feed Efficiency. In: Feed Efficiency in the Beef Industry, edited by Hill RAWiley-Blackwell, 2012, p. 251-273. 12. Bryson JM, Phuyal JL, Swan V, and Caterson ID. Leptin has acute effects on glucose and lipid metabolism in both lean and gold thioglucose-obese mice. Am J Physiol Endocrinol Metab 277: E417-422, 1999. 13. Buchanan FC, Fitzsimmons CJ, Van Kessel AG, Thue TD, Winkelman-Sim DC, and Schmutz SM. Association of a missense mutation in the bovine leptin gene with carcass fat content and leptin mRNA levels. Genet Sel Evol 34: 105-116, 2002. 14. Carstens GE, Theis CM, White MB, Welsh TH, Jr., Warrington BG, Randel RD, Forbes TDA, Lippke H, Greene LW, and Lunt DK. Residual feed intake in beef steers: I. Correlations with performance traits and ultrasound measures of body composition. Proceedings of the Western Section Meeting, American Society of Animal Science 53: 552-555, 2002. 15. Castro Bulle FCP, Paulino PV, Sanches AC, and Sainz RD. Growth, carcass quality, and protein and energy metabolism in beef cattle with different growth potentials and residual feed intakes. J Anim Sci 85: 928-936, 2007. 16. Ceddia RB, William WN, Jr., and Curi R. The response of skeletal muscle to leptin. Front Biosci 6: D90-97, 2001. 17. Challiss R, and Ferre P. Integration of carbohydrate and lipid metabolism in skeletal muscel during postnatal development. Reproductive Nutritional Development 28: 805-815, 1988. 18. Ciccioli NH, Wettemann RP, Spicer LJ, Lents CA, White FJ, and Keisler DH. Influence of body condition at calving and postpartum nutrition on endocrine function and reproductive performance of primiparous beef cows. J Anim Sci 81: 3107-3120, 2003. 19. Crews DH, Jr. , Lowerison M, Caron N, and Kemp RA. Genetic parameters among growth and carcass traits of Canadian Charolais cattle. Canadian Journal of Animal Science 84: 589-597, 2004. 20. Davis ME, and Simmen RC. Genetic parameter estimates for serum insulin-like growth factor-I concentration and carcass traits in Angus beef cattle. Journal of Animal Science 78: 2305-2313, 2000. 21. Davis ME, Wick MP, and Maquivar MG. Hormonal Regulation of Feed Efficiency. In: Feed Efficiency in the Beef Industry, edited by Hill RAWiley-Blackwell, 2012, p. 225-250. 22. Duan C, Ren H, and Gao S. Insulin-like growth factors (IGFs), IGF receptors, and IGF-binding proteins: Roles in skeletal muscle growth and differentiation. General and Comparative Endocrinology 167: 344-351, 2010. 23. Eguinoa P, Brocklehurst S, Arana A, Mendizabal JA, Vernon RG, and Purroy A. Lipogenic enzyme activities in different adipose depots of Pirenaican and Holstein bulls and heifers taking into account adipocyte size. J Anim Sci 81: 432-440, 2003. 24. Exton SC, Herd RM, and Arthur PF. Identifying bulls superior for net feed intake, intramuscular fat and subcutaneous fat. Animal Production in Australia 25: 57-60, 2004. 25. Fitzsimmons CJ, Schmutz SM, Bergen RD, and McKinnon JJ. A potential association between the BM 1500 microsatellite and fat deposition in beef cattle. Mamm Genome 9: 432-434, 1998. 26. Florini JR, Ewton DZ, and Coolican SA. Growth Hormone and the Insulin-Like Growth Factor System in Myogenesis. Endocrine Reviews 17: 481-517, 1996. 27. Griffin ME, Marcucci MJ, Cline GW, Bell K, Barucci N, Lee D, Goodyear LJ, Kraegen EW, White MF, and Shulman GI. Free fatty acid-induced insulin resistance is associated with activation of protein kinase C theta and alterations in the insulin signaling cascade. Diabetes 48: 1270-1274, 1999. 28. Herd RM, Archer JA, and Arthur PF. The effect of divergent selection for yearling growth rate on growth at pasture and final size of Angus steers. In: Breeding responding to client needs Association for the Advancement of Animal Breeding and Genetics Proceedings of the Twelfth Conference, Dubbo, NSW, Australia 6th-10th April 1997: Part 11997, p. 323-327. 29. Herd RM, Archer JA, Arthur PF, Richardson EC, Wright JH, Dibley KCP, and Burton DA. Performance of progeny of high vs. low net feed conversion efficiency cattle. In: Breeding responding to client needs Association for the Advancement of Animal Breeding and Genetics Proceedings of the Twelfth Conference, Dubbo, NSW, Australia 6th-10th April 1997: Part 21997. 30. Herd RM, and Arthur PF. Physiological basis for residual feed intake. J Anim Sci 87: E64-71, 2009. 31. Hill RA, and Ahola JK. Feed Efficiency Interactions with Other Traits: Growth and Product Quality. In: Feed Efficiency in the Beef Industry, edited by Hill RAWiley-Blackwell, 2012, p. 145-158. 32. Hill RA, Dunshea FR, and Dodson MV. Growth and Livestock. In: Biology of Growth of Domestic Animals, edited by Scanes CG. Ames: Iowa State Press, 2003. 33. Hood DA. Invited Review: Contractile activity-induced mitochondrial biogenesis in skeletal muscle. Journal of Applied Physiology 90: 1137-1157, 2001. 34. Hood DA, Irrcher I, Ljubicic V, and Joseph A-M. Coordination of metabolic plasticity in skeletal muscle. Journal of Experimental Biology 209: 2265-2275, 2006. 35. Hoppeler H. Exercise-induced ultrastructural changes in skeletal muscle. International journal of sports medicine 7: 187-204, 1986. 36. Hutley LJ, Herington AC, Shurety W, Cheung C, Vesey DA, Cameron DP, and Prins JB. Human adipose tissue endothelial cells promote preadipocyte proliferation. Am J Physiol Endocrinol Metab 281: E1037-E1044, 2001. 37. Kerley MS. Nutrition and Feed Efficiency of Beef Cattle. In: Feed Efficiency in the Beef Industry, edited by HIll RAWiley-Blackwell, 2012, p. 75-92. 38. Kokta TA, Dodson MV, Gertler A, and Hill RA. Intercellular signaling between adipose tissue and muscle tissue. Domestic Animal Endocrinology 27: 303-331, 2004. 39. Kolath WH, Kerley MS, Golden JW, Shahid SA, and Johnson GS. The relationships among mitochondrial uncoupling protein 2 and 3 expression, mitochondrial deoxyribonucleic acid single nucleotide polymorphisms, and residual feed intake in Angus steers. J Anim Sci 84: 1761-1766, 2006. 40. Kuang S, and Rudnicki MA. The emerging biology of satellite cells and their therapeutic potential. Trends in Molecular Medicine 14: 82-91, 2008. 41. Lebon V, Dufour S, Petersen KF, Ren J, Jucker BM, Slezak LA, Cline GW, Rothman DL, and Shulman GI. Effect of triiodothyronine on mitochondrial energy coupling in human skeletal muscle. The Journal of Clinical Investigation 108: 733-737, 2001. 42. Lund PK, Moats-Staats BM, Hynes MA, Simmons JG, Jansen M, D'Ercole AJ, and Van Wyk JJ. Somatomedin-C/insulin-like growth factor-I and insulin-like growth factor-II mRNAs in rat fetal and adult tissues. Journal of Biological Chemistry 261: 14539-14544, 1986. 43. May SG, Savell JW, Lunt DK, Wilson JJ, Laurenz JC, and Smith SB. Evidence for preadipocyte proliferation during culture of subcutaneous and intramuscular adipose tissues from Angus and Wagyu crossbred steers. J Anim Sci 72: 3110-3117, 1994. 44. Meyer AM, Kerley MS, and Kallenbach RL. The effect of residual feed intake classification on forage intake by grazing beef cows. J Anim Sci 86: 2670-2679, 2008. 45. Miller MF, Cross HR, Lunt DK, and Smith SB. Lipogenesis in acute and 48-hour cultures of bovine intramuscular and subcutaneous adipose tissue explants. J Anim Sci 69: 162-170, 1991. 46. Minokoshi Y, Kim YB, Peroni OD, Fryer LG, Muller C, Carling D, and Kahn BB. Leptin stimulates fatty-acid oxidation by activating AMP-activated protein kinase. Nature 415: 339-343, 2002. 47. Montague CT, Prins JB, Sanders L, Digby JE, and O' Rahilly S. Depot- and sex-specific differences in human leptin leptin mRNA expression: Implications for the control of regional fat distribution. Diabetes 46: 342-347, 1997. 48. Muoio DM, Dohm GL, Fiedorek FT, Tapscott EB, and Coleman RA. Leptin directly alters lipid partitioning in skeletal muscle. Diabetes 46: 1360-1363, 1997. 49. Muoio DM, Dohm GL, Tapscott EB, and Coleman RA. Leptin opposes insulin's effects on fatty acid partitioning in muscle isolated from obese ob/ob mice. Am J Physiol 276: E913-E921, 1999. 50. Murphy LJ, Bell GI, and Friesen HG. Tissue Distribution of Insulin-Like Growth Factor I and II Messenger Ribonucleic Acid in the Adult Rat. Endocrinology 120: 1279-1282, 1987. 51. Niesler CU, Prins JB, O' Rahilly S, Siddle K, and Montague CT. Adipose depot-specific expression of cIAP2 in human preadipocytes and modulation of expression by serum factos and TNFa. International Journal of Obesity 25: 1027-1033, 2001. 52. Nkrumah JD, Basarab JA, Wang Z, Li C, Price MA, Okine EK, Crews DH, Jr., and Moore SS. Genetic and phenotypic relationships of feed intake and measures of efficiency with growth and carcass merit of beef cattle. J Anim Sci 85: 2711-2720, 2007. 53. Nkrumah JD, Li C, Basarab JB, Guercio S, Meng Y, Murdoch B, Hansen C, and Moore SS. Association of a single nucleotide polymorphism in the bovine leptin gene with feed intake, feed efficiency, growth, feeding behaviour, carcass quality and body composition. Canadian Journal of Animal Science 84: 211-219, 2004. 54. Oddy VH, and Owens PC. Insulin-like growth factor I inhibits degradation and improves retention of protein in hindlimb muscle of lambs. American Journal of Physiology - Endocrinology And Metabolism 271: E973-E982, 1996. 55. Oksbjerg N, Gondret F, and Vestergaard M. Basic principles of muscle development and growth in meat-producing mammals as affected by the insulin-like growth factor (IGF) system. Domestic Animal Endocrinology 27: 219-240, 2004. 56. Philippou A, Halapas A, Maridaki M, and Koutsilieris M. Type I insulin-like growth factor receptor signaling in skeletal muscle regeneration and hypertrophy. J Musculoskelet Neuronal Interact 7: 208-218, 2007. 57. Richardson EC, Archer JA, Arthur PF, Thompson JM, Herd RM, and Oddy VH. Body composition and implications for heat production and Angus steer progeny of parents selected for and against residual feed intake. Australian Journal of Experimental Agriculture 41: 1065-1072, 2001. 58. Richardson EC, and Herd RM. Biological basis for variation in residual feed intake in beef cattle. 2. Synthesis of results following divergent selection. Australian Journal of Experimental Agriculture 44: 431-440, 2004. 59. Richardson EC, and Herd RM. Biological basis for variation in residual feed intake in beef cattle. 2. Synthesis of results following divergent selection. Australian Journal of Experimental Agriculture 44: 431-440, 2004. 60. Richardson EC, Herd RM, Archer JA, and Arthur PF. Metabolic differences in Angus steers divergently selected for residual feed intake. Australian Journal of Experimental Agriculture 44: 441-452, 2004. 61. Richardson EC, Herd RM, Archer JA, and Arthur PF. Metabolic differences in Angus steers divergently selected for residual feed intake. Australian Journal of Experimental Agriculture 44: 441-452, 2004. 62. Richardson EC, Herd RM, Archer JA, Woodgate RT, and Arthur PF. Steers bred for improved net feed efficiency eat less for the same feedlot performance. In: Animal Production in Australia Proceedings of the Australian Society of Animal Production1998. 63. Roden M, Price TB, Perseghin G, Petersen KF, Rothman DL, Cline GW, and Shulman D. Mechanism of free fatty acid-induced insulin resistance in humans. Journal of Clinical Investigation 97: 2859-2865, 1996. 64. Salmon WDJ, and Daughaday WH. A hormonally controlled serum factor which stimulates sulfate incorporation by cartilage in vitro. J Lab Clin Med 49: 825-836, 1957. 65. Schenkel FS, Miller SP, and Wilton JW. Genetic parameters and breed differences for feed efficiency, growth, and body composition traits of young beef bulls. Canadian Journal of Animal Science 84: 177-185, 2004. 66. Solinas G, Summermatter S, Mainieri D, Gubler M, Pirola L, Wymann MP, Rusconi S, Montani JP, Seydoux J, and Dulloo AG. The direct effect of leptin on skeletal muscle thermogenesis is mediated by substrate cycling between de novo lipogenesis and lipid oxidation. FEBS Lett 577: 539-544, 2004. 67. Thornton KJ, Welch CM, Davis LC, Doumit ME, Hill RA, and Murdoch GK. Bovine sire selection based upon maintenance energy impacts muscle fiber type and meat color of F1 progeny. Journal of Animal Science 2012. 68. Trayhurn P, and Beattie JH. Physiological role of adipose tissue: white adipose tissue as an endocrine and secretory organ. Proc Nutr Soc 60: 329-339, 2001. 69. Van Harmelen V, Dicker A, Ryden M, Hauner H, Lonnqvist F, Naslund E, and Arner P. Increased Lipolysis and Decreased Leptin Production by Human Omental as Compared With Subcutaneous Preadipocytes. Diabetes 51: 2029-2036, 2002. 70. Welch CM, Ahola JK, Hall JB, Murdoch GK, Crews DH, Davis LC, Doumit ME, Price WJ, Keenan LD, and Hill RA. Relationships among performance, residual feed intake, and product quality of progeny from Red Angus sires divergent for maintenance energy EPD. Journal of Animal Science 2012. 71. Welch CM, McGee M, Kokta TA, and Hill RA. Muscle and Adipose Tissue: Potential Roles in Driving Variation in Feed Efficiency. In: Feed Efficiency in the Beef Industry, edited by Hill RAWiley-Blackwell, 2012, p. 175-198. 72. Yakar S, Liu J-L, Stannard B, Butler A, Accili D, Sauer B, and LeRoith D. Normal growth and development in the absence of hepatic insulin-like growth factor I. Proceedings of the National Academy of Sciences 96: 7324-7329, 1999. 73. Yu HY, Inoguchi T, Kakimoto M, Nakashima N, Imamura M, Hashimoto T, Umeda F, and Nawata H. Saturated non-esterified fatty acids stimulate de novo diacylglycerol synthesis and protein kinase c activity in cultured aortic smooth muscle cells. Diabetologia 44: 614-620, 2001. 74. Zhang Y, Guo KY, Diaz PA, Heo M, and Leibel RL. Determinants of leptin gene expression in fat depots of lean mice. Am J Physiol Regulatory Integrative Compl Physiol 282: 226-234, 2001.