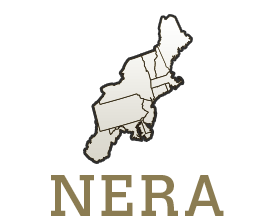
NE1045: Design, Assessment, and Management of Onsite Wastewater Treatment Systems: Addressing the Challenges of Climate Change
(Multistate Research Project)
Status: Inactive/Terminating
NE1045: Design, Assessment, and Management of Onsite Wastewater Treatment Systems: Addressing the Challenges of Climate Change
Duration: 10/01/2010 to 09/30/2015
Administrative Advisor(s):
NIFA Reps:
Non-Technical Summary
Statement of Issues and Justification
Onsite wastewater treatment systems (OWTS; widely known as "septic systems", or decentralized wastewater treatment systems) serve approximately 25 percent of the United States population. In rural and unsewered watersheds, they are the only means to treat wastewater. As federal subsidies for funding centralized municipal treatment have ended, new development in rural areas is almost exclusively dependant upon OWTS. Typically these systems serve individual rural residences and small farmsteads, but may also treat flows from small community cluster treatment systems. New challenges necessitate advances in our understanding, design and management of OWTS that warrant a multi-state effort of Land Grant scientists. Of particular note are three drivers of change:
1) Recognition that OWTS are no longer a temporary, 'stop gap' approach that will be replaced by municipal sewers. OWTS are increasingly in use for decades and are used for all types of rural establishments - raising concerns for long-term hydraulic and treatment performance.
2) Increasing demands by public and environmental health professionals that OWTS achieve high and reliable levels of nitrogen, phosphorus, emerging chemicals and pathogen removal rates - well beyond those associated with conventional technologies.
3) Challenges presented by the possible decadal changes in climate, such as rising water tables or severe drought, argue for new approaches to site selection, design and water reuse.
As with any technical field, the science must continually evolve to produce site suitability predictive tools, technologies and management policies that meet changing societal needs and are responsive to climate change and national water requirements, while protecting public health and the environment. In addition, policies must address the challenges that result from implementation of the new solutions. While, conventional OWTS appear deceptively simple, consisting of a solids storage tank and a soil absorption field for effluent. The vast variability in climate, soils, hydrology, use and environmental sensitivity of rural America requires the application of soil physics, soil microbiology, soil chemistry, pedology, hydrology and engineering to site, design and manage systems that will protect human and environmental health and still encourage rural development. Beyond science and technology, advances in operation, maintenance, and management of OWTS at the watershed, county and state level are an essential component in maintaining the functionality of these systems. Thus, a multi-disciplinary effort is required to assure treatment capability and build greater confidence at all user levels. The outcome of this work will contribute to the many aspects of rural life that are linked to OWTS including economic viability and environmental and human health.
Antiquated, poorly sited, or improperly designed onsite wastewater systems degrade public and environmental health through delivery of pathogens and nutrients. In the past, OWTS were considered a temporary fix until sewer extensions were installed, and system maintenance and management were often ignored. Today, there is a steady paradigm shift within the field. Around the nation, these older systems are now being replaced by state-of-the-science technologies that incorporate management as a key long-term operations component. This is occurring more readily in some states than in others. For instance in Rhode Island, 31 percent of the population uses onsite wastewater systems, and innovative and alternative onsite wastewater treatment systems currently account for about 27 percent of all system permit applications approved by the state regulatory agency (RIDEM, 2008a); and several critical resource area watersheds require mandatory nitrogen removal OWTS technology be used (RIDEM, 2008b).
Because they disperse treated wastewater in the same hydrologic basins where groundwater is extracted, OWTS help address the growing challenges of water shortages by replenishing in-basin aquifers, wetlands, rivers and lakes. This recharge helps to maintain base flows to interconnected ground and surface waters and provides critical supplies for down gradient water users and for water shortage and drought abatement. Advanced OWTS offer the potential for reuse of treated wastewater that can conserve potable water supplies of a rural community by utilizing properly treated wastewater for toilet flushing and biomass, crop and landscape irrigation. For this to happen safely and effectively, residents and decision makers must have knowledge of tested, reliable technologies that address the particular suite of watershed, site, soil and climatic constraints (and projected climatic change) in a given area. In addition, private and public sector wastewater professionals must be well-versed in the application of these technologies. With continued research and improvements in development, assessment and management, OWTS can be a permanent part of the US rural infrastructure, providing safe, sustainable, and economical treatment along with wastewater reuse, recycling, aquifer recharge and surface water replenishment.
Advances in siting and design for OWTS can mitigate the risks associated with intense weather extremes attributed to climate change. Watershed and individual site characteristics can change in response to storm-related and long-term climate change conditions. In some areas, climate change is predicted to generate wetter soils and higher water tables, due to cooler and wetter dormant seasons, sea level rise and more extensive flooding in response to increased, high intensity storms (Hayhoe et al., 2006). This is especially critical for low lying regions with little topographic relief (e.g. coastal plains subject to sea level rise) or areas where soils have low porosity - locations where drainage is already challenging. Soil wetness and water table depth are key factors that strongly influence OWTS suitability - and current siting and design practices only rely on current and historic conditions. Better site suitability tools are needed to determine critical watershed and site factors susceptible to climate change, identify locations where they occur and predict and model how these environs will respond to the possible range of changing climatic conditions over the 30-50 years that OWTS are likely to be in use. Having robust state-of-the-science advanced OWTS technologies available that can function and adapt to changing high risk conditions, and cope with and mitigate the effects of climatic perturbations, helps assure that water quality is maintained for all down gradient users.
In their response to Congress, the US Environmental Protection Agency cited many of the afore-mentioned factors as benefits to using decentralized or onsite wastewater treatment systems (USEPA, 1997). However, among the barriers to more widespread implementation of decentralized systems the EPA cited the following:
a. Lack of knowledge and public misperception about onsite wastewater systems.
b. Lack of management programs.
c. Financial barriers.
Although considerable effort has been expended to overcome these barriers during the past decade, continued work is needed to maintain momentum and achieve more widespread acceptance of this technology. Certain regions of the United States have implemented progressive revisions to regulatory codes, whereas others have moved forward only minimally. Where change in the OWTS field has occurred it has been spurred on by advances in treatment technologies, research in onsite wastewater treatment processes and management, as well as education of wastewater practitioners, land use planners, developers and regulators.
Historically, onsite wastewater research and outreach education began with work conducted through Agricultural Experiment Stations (AES) at Land Grant Universities. This targeted the rural communities where Cooperative Extension had a strong outreach presence. The Land Grant professionals effecting this change were mainly from agricultural engineering and soil science backgrounds. The objective of this research-based outreach education was to make improvements in onsite wastewater infrastructure to help make rural landscapes more sustainable.
The expertise of the Land Grant University System continues to be sought out by rural stakeholders who recognize the importance of research on new onsite wastewater technology designs and treatment performance. The application of this research base into high quality, non-biased, standardized training materials is essential to raise the knowledge levels of clientele and influence change of practice.
Until this current proposal, a formal multi-state project did not exist to coordinate OWTS research within the Land Grant System. Existing efforts have been informally organized through the Consortium of Institutes for Decentralized Wastewater Treatment (CIDWT), an organization of Land Grant institutions, other training entities, and industry and agency partners dedicated to decentralized wastewater system research, education, outreach and training (http://www.onsiteconsortium.org). Land Grant System institutions represent the core of CIDWT, and play a key role in providing third party, non-biased research and science-based educational and training materials for CIDWT members.
Several Land Grant System institutions have established state or regional onsite wastewater training centers which serve to provide technical information to wastewater practitioners and continuing education credit classes for professional license renewals. This is a ready and established means for technical transfer of data and outreach materials that are generated.
The members of our proposed Multi-State Hatch Project have strong contacts with industry partners (such as the National Onsite Wastewater Recycling Association, National Environmental Health Association, National Association of Wastewater Transporters) and government agencies (specifically USEPA) to assure that research and educational issues important to wastewater practitioners are addressed. USEPA has funded many OWTS demonstration projects, capacity building grant projects, and curriculum development efforts. In several instances, Land Grant System universities have functioned as ad hoc work teams to spearhead these extramural EPA grant projects to generate research and disseminate emerging information to a wide variety of onsite wastewater system user groups. This proposal will formalize these efforts into an all-encompassing AES collaborative project that will solidify the leadership role of Land Grant Institutions in OWTS and continue the momentum generated by ad hoc efforts.
The enormous variation in soils, geology, climate and hydrology that affect the proper function of onsite wastewater treatment systems across the nation requires multi-regional collaboration to ensure standardization of state-of-the-science and engineering approaches to assessment and protocols. A diverse working team of university scientists, along with industry, regulatory agency personnel and private sector wastewater practitioner partners has been assembled through the CIDWT framework. This will afford considerable momentum to the creation of a successful AES multi-regional model.
Hypothesis: Current OWTS will fail in light of climate change. With predicted climate change, climate variability will be exacerbated by periods of very wet and very dry hydrologic conditions. This will necessitate reevaluation of the current design guides and parameters for OWTS. Without new design criteria the current systems have potential to fail.
Related, Current and Previous Work
Regulations related to site and soil suitability for onsite wastewater treatment have evolved since the early 1970s to place less reliance upon the "percolation (perc) test" and greater emphasis on soil morphological and landscape properties as well as site evaluation methods. Several studies have integrated soil morphologic factors to help determine soil long-term acceptance rates for wastewater and site and soil suitability (Bouma, 1975; Tyler et al., 1991; Tyler and Converse, 1994; Brown et al., 1994; Miles and West, 2001; Tyler, 2001; Lindbo et al., 2007). Other studies have focused on the relationship between soil morphology, water table fluctuation and duration (Stolt et al., 2001; Morgan, 2002; Morgan and Stolt, 2006). New field and lab procedures need to be developed and evaluated that recognize high-risk conditions for soil treatment, such as, areas at risk for rising water tables or partial saturation due to climate change, or sites with constraints for treating high strength wastewater.
Decentralized OWTS have been shown to contribute to groundwater and surface water contamination, especially in areas with dense development (Gold et al., 1990; Bouchard, et al., 1992; Postma et al., 1992; Gold and Sims, 2000; Hallberg, 1989; Hantzsche and Finnemore, 1992; Rich, 2005). Conventional OWTS remove 0 - 30 percent of the total nitrogen load from residential wastewater (Keeney, 1986; Siegrist and Jenssen, 1989; Lamb et al., 1990; Kaplan, 1991; USEPA, 2002). This issue has become most acute in shallow, sole-source aquifers and in coastal regions where poorly flushed lagoons and ponds tend to accumulate nitrogen and pathogens, impairing water quality and shellfish and finfish production and harvesting (Ryther and Dunstan, 1971; Weiskel and Howes, 1992). The amount of contaminants entering surface waters that are contributed by onsite wastewater treatment systems varies widely. In the Buttermilk Bay watershed, a poorly flushed eastern Massachusetts coastal lagoon, an estimated 74% of annual nitrogen loading to the Bay is provided by onsite wastewater treatment systems (Horsley Whitten Hegeman, Inc., 1991). Whereas, the percentage of N from OWTS entering three major Delaware Inland Bays ranged from 11 to 16% (Ritter, 1986). Although scant data exists on OWTS phosphorus contamination of surface water, Robertson et al. (1998) documented P migration in groundwater from OWTS fields adjacent to lake shore cottages in Canada. A mid-west rural lakes study estimated 2 to 10% of the total-P loading to the lakes were from OWTS (USEPA, 1983). Viral transport from OWTS has been shown to vary considerably, with horizontal distances of 35, 67, and 183 meters reported in the literature (Scandura and Sobsey, 1977; Vaughn et al., 1983; and Schaub and Sorber, 1977, respectively). To help address this problem, various advanced onsite wastewater treatment technologies have been developed and evaluated for treatment performance (Lamb et al., 1990, 1991; Whitmeyer, et al. 1991; Gold et al., 1992; Emerick et al., 1997; Loomis et al., 2001, 2004; Obropta and Berry, 2005; Rich, 2005; Amador et al., 2007). Research into new, advanced treatment technologies must continue to assure that emerging science is keeping pace with the growing challenges of climate change and the need for a clean and plentiful supply of water to sustain rural America.
Objectives
-
Develop a better understanding of the relationship between OWTS design features and the soil transport parameters (e.g., porosity, hydraulic conductivity, residual moisture content).
-
Develop new design criteria for OWTS as it relates to climate change for the purposes of adaptation and mitigation.
-
Examine the new design criteria for diverse soils, geomorphology, topography, and climate conditions.
-
Develop educational materials and tools to acquaint the public and practitioners to management, operation, maintenance and health issues related to OWTS in light of adaptation to climate change.
Methods
The methods used in a multi-state project, where there are many regulatory jurisdictions, are complex. The breadth and depth of the following methods will directly reflect the level of funding from participating AESs and outside sources and may change as more participants join the project.Objective 1. Develop a better understanding of the relationship between OWTS design features and the soil hydraulic transport parameters (e.g., porosity, hydraulic conductivity, residual moisture content).
Since all systems rely on the hydraulic properties of the soil for dispersal and treatment it is important to relate these properties to system design.
Hydraulic Conductivity and Soil Porosity
In-situ hydraulic conductivity will be measured in triplicate in major horizons of selected soils. Procedures and data analysis will follow standard methods (Amoozegar, 2004; Amoozegar and Wilson, 1999).
For laboratory measurement of saturated hydraulic conductivity intact soil cores will be collected from different horizons from selected soils. The horizon/depth of sampling will match the depth where in situ Ksat measurements will be conducted. The samples will be collected by either a Uhland type sampler or by a hydraulic sampler. Any sample collected by a sampling tube will be covered with paraffin and prepared and analyzed for hydraulic conductivity measurements by the procedure described by Amoozegar (1988) and Amoozegar and Wilson (1999). The flow rate through the column will be measured with time and a Ksat value will be calculated by Darcys law for each measurement.
At the termination of Ksat measurements, all intact cores will be analyzed for water retention, also known as water characteristics (Dane and Hopmans, 2002). Each intact core will be placed in a Buchner funnel and resaturated. The funnel will then be sealed and air pressure equivalent to -10, -50, -100, and -300 cm soil water pressure heads will be applied to the saturated soil core in steps. The volume of water drained from each core under each pressure step will be measured. Following the last pressure head application, the core will be removed from the funnel and its water content determined by weighing the wet soil, drying it in an oven at 105 oC, weighing the oven-dried soil, and determining the amount of water lost from the wet core. Mass of oven-dried soil and volume of the core will be used to determine the soil bulk density. Based on the volumetric water content of the core after the last pressure head application, the amount of water that was held in the soil at saturation (zero pressure head) and all other pressure heads will be determined. Bulk sample from each oven-dried soil will be used in a pressure plate apparatus to determine its water holding capacity at 1-, 5-, and 15-bar pressure for constructing a complete soil water characteristic curve for that soil sample. The volumetric water content at saturation will be taken to be equivalent to the soil total porosity.
Residual Water Content
With cooperation from health departments in selected counties a number of OWTS with subsurface dispersal STAs will be selected for monitoring/ sampling. The soils in the STA of each system will be characterized in the field by hand auger boring. Soil samples from different horizons and locations within each STA will be collected and analyzed for complete characterization. Soil water content at five different depth intervals at three locations within each of six STAs will be measured 2 to 4 times a month by a PR2 probe system (Dynamax, TX).
Fate and Transport
Intact soil core samples and bulk soil materials from the soils used for hydraulic conductivity and porosity determinations, as mentioned above, will be collected for laboratory studies. Subsamples from the bulk soil materials will be analyzed for particle size distribution, pH, CEC, N, P, basic cation content, and other pertinent properties. Attenuation capacity of each soil material for N and P will be assessed by a batch study, and their transport through the soils will be evaluated by a column experiment. For the batch study, equivalent to 20 g of oven-dry subsamples of each soil material will be mixed thoroughly with 50 mL of a solution containing ammonium nitrate (NH4NO3) and potassium phosphate (K3PO4) at different concentrations. The amount adsorbed will be regressed against their respective concentration in soil solution to obtain adsorption isotherms for each soil-nutrient combination. The intact core samples will be used for a lab column experiment using a NH4NO3-K3PO4 solution. Breakthrough curves for K, NH4, NO3, and P will be obtained and a one-dimensional solute transport model will be fitted to the breakthrough curves to determine the diffusion/dispersion coefficient and retardation factor for each element (or ion).
Modeling
Both simulation models and monitoring methods will be used to accomplish our objectives. Using simulation models, we will optimize the OWTS design by adjusting the design parameters and looking at the model output in terms of discharge capacity, chemical discharge, and pathogen fate. Once we have identified one or two optimal design criteria through simulation, we will test those designs in field-based pilot studies and collect data to show the workability of the new design criteria.
Two models will be used that represent different levels of complexity. As a relatively simple model, the STUMOD (Soil Treatment Unit Model; McCray et al., 2010) will be employed. STUMOD input parameters include effluent concentrations and hydraulic loading rates. The output is the expected steady-state performance (i.e., constituent concentration) at the center under the point of effluent application. STUMOD was developed for transport in the unsaturated zone, which is assumed to be predominantly vertical flow with contaminants transported mainly by advection and the effect of dispersion ignored. When the effluent application rate is constant, the infiltration reaches steady state and the pressure profile or soil moisture profile does not change with time. The effect of moisture content on nitrification and denitrification is calculated based on soil moisture profile. STUMOD accounts for the effect of temperature on nitrification and denitrification.
As a more complex model, a modified version of HYDRUS(2D/3D) (`imonek et al., 2006) will be used. HYDRUS is a numerical model that simulates water, solute, and heat transport in soil. We will use a 2D simulation that will be appropriate for STA trench, low pressure pipe (LPP), pressurized shallow narrow drainfield (PSND), and drip irrigation systems. The model will simulate the movement and transformations of different forms of N (based on work done at the University of Georgia; McCray et al., 2010) and the movement and transformations of viruses (based on the work done at University of Rhode Island; McCray et al., 2010). HYDRUS does not assume steady state flow and will therefore respond to rapid changes in moisture and temperature, as well as the long- term changes in these variables. Also, the effect of modified systems such as LPP and PSND will be tested. HYDRUS input parameters include soil hydraulic properties, daily weather, and concentrations of effluent from the septic tank or advanced treatment unit. Outputs are predicted concentrations of N and viruses moving below a given soil depth.
Objective 2. Develop new design criteria for OWTS as it relates to climate change for the purposes of adaptation and mitigation.
The overarching goal of this objective is to research emerging OWTS technologies and improve current treatment processes to maximize system robustness, enabling systems to cope with climate change and the wide variations that already exist in US soils, climate, geology and hydrology.
With predicted climate change, variability will likely be exacerbated by prolonged periods of very wet and very dry hydrologic conditions. Population growth is expanding water demands in water poor regions, droughts are worsening, while at the same time drinking water supplies are dwindling and water costs are increasing. To address these issues we propose to investigate the technological challenges of wastewater reuse for toilet flushing and irrigating crops, biomass and landscapes. More widespread use of these practices would help alleviate local water shortages while recycling wastewater nutrients, thereby placing less demand on local potable water supplies and helping to reduce costs for commercial fertilizers.
Reliable treatment performance is critical for effective wastewater reuse. We will study the treatment performance of OWTS technologies in various regions of the US. Approved and emerging technologies will be selected and monitored for treatment and operational performance under various geologic, soil, climatic and hydrologic conditions. Working with regulatory partners, study sites will be selected and homeowner access permission secured. Temperature influences on treatment performance and function will be evaluated by monitoring systems during warm and cold seasons. The influence of seasonal home occupancy on treatment performance will be evaluated by monitoring start-up and active use periods for seasonally-used systems (typically found in ocean front and lakeside shoreline areas). Wastewater samples will be collected before and after technology components to assess contaminant concentrations and percent reduction.
Wastewater samples will be analyzed for DO, temperature and hydraulic flow in the field and for typical wastewater parameters in the lab (BOD, TSS, pH, alkalinity, TP, TN and fecal coliform; APHA, 1998). We will work with our regulatory and technology manufacturer partners to utilize results of our research to modify technology design and operational parameters to maximize treatment performance.
As noted in Attachment A, wastewater typically flows though a conventional OWTS by gravity. Gravity dispersal in a STA limits the potential treatment of wastewater and poses challenges to using treated wastewater for landscape, biomass or crop irrigation. In contrast, use of PSNDs showed a 33 to 73 percent reduction in residual N levels from advanced treatment system effluent (Holden at al., 2004). With this in mind, we will research the use of alternative wastewater dispersal methods that would promote upper soil moisture management, recycling and sequestration of nutrients and carbon and unsaturated wastewater flow. To accomplish this, we will evaluate the use of LPP, PSND and drip irrigation to disperse wastewater into shallow placed STAs where biochemical reactivity is expected to be high and where plant roots will be in contact with effluent wetting fronts. Comparisons of different dispersal methods will be conducted under different US climate, soil, geologic and hydrologic conditions. Suction cup lysimeters will be placed at 30 and 90 cm beneath the STA and at similar points in control areas (Holden at al., 2004). Soil pore water collected in the lysimeters will be analyzed for TP and TN. Top growth of biomass will be monitored within control and STA dispersal areas.
OWTS rely on biochemical processes to renovate wastewater. To maximize treatment potential we will improve our understanding of the relationship among microorganisms, biogeochemical processes, treatment mechanisms and transformations of pollutants, physiochemical conditions and antimicrobial compounds on treatment performance.
We will focus our efforts on (i) understanding the structure and function of microbial communities and groups of microorganisms in treatment processes and (ii) characterizing their responses to environmental challenges. Micro- and mesocosm-scale experiments mimicking OWTS conditions will be conducted to examine the dynamics of microbial populations and biogeochemical processes in STAs (e.g. Potts et al., 2004; Amador et al., 2006). This information will be used to test hypotheses on the interplay among physicochemical parameters, microbial ecology and biogeochemistry of OWTS.
We will characterize microbial populations and communities, with emphasis on molecular genetics methods. Community structure will be examined using PCR, cloning and sequencing of targeted areas of the rRNA subunits of fungi and bacteria (Tomaras et al., 2009) as well as terminal restriction fragment length polymorphism (TRFLP) methodology (Kraighera et al., 2008). We will also use gene probes targeted to particular processes (e.g. ammonia oxidation, methanogenesis) alone and in combination with fluorescence in situ hybridization methods (FISH) (Kim et al., 2004) to follow dynamics of groups of microorganisms involved in renovation in STAs. Organisms in the domains Archeae, Bacteria and Eukaryota (fungi) will be targeted. These analyses will identify organisms present in treatment areas, their potential function, and their response to environmental challenges.
To examine biogeochemical treatment processes, we will employ a variety of approaches, including: (i) measurement of changes in concentration of electron acceptors and donors using conventional methods (APHA, 1998), (ii) use of stable isotope-labeled compounds (e.g. 13C-antibiotics, 15N-NH4, 34S-SO4) to track their fate, and (iii) changes in the concentration of mRNA coding for enzymes involved in transformations of interest. This information, in combination with data on the structure and dynamics of microbial populations, will provide us with a picture of the ecology of biogeochemical processes that improve the quality of water in STAs to address the challenges posed by climate change.
To determine the effect climate change may have on OWTS model simulations, weather scenarios that are being developed using regional climate models (RCM) that downscale global climate model predictions to regions will be used as they become available (Patz et al., 2005). These weather scenarios will be used to drive the STUMOD and HYDRUS models over a simulation period of one year and compared to a simulation using a year that represents the current climate.
Objective 3. Examine the new design criteria for diverse soils, geomorphology, topography, and climate conditions.
Current and new designs need constant tests to evaluate their effectiveness in different regions. In order to make the regional results comparable across the country, standard site selection and evaluation tools need to be established and followed. Once this is done, surveys of existing systems, soils and sites will be used to evaluate current design criteria. In order to evaluate new design criteria siting models will need to be used. All models must be calibrated to existing soil and site conditions in order to be valid.
Site Selection Methods
Study areas will be chosen to represent typical landscapes and soils in a given region. Preliminary investigation using Web Soil Survey (http://websoilsurvey.nrcs.usda.gov/app/HomePage.htm) combined with reconnaissance on the site will confirm the soil series.
Field Methods
Transects will be located in the forest or inactive grassland/forest edge, and will be located to cross from well drained through poorly drained soils as applicable. Locations for observation wells, piezometers and redox probes (as applicable) will be determined through reconnaissance boring and will be installed following standard procedures. Wells will extend to approximately 80 cm, to the depth of seasonal low water table, or to a restrictive horizon contact (lithic, fragipan, etc.) whichever is shallower. Piezometers and redox probes will be placed at several depths spanning the range (±30 cm) of expected seasonal high water table. In addition to wells with water level recorders, a control/check well will be installed. A recording rain gauge will also be installed on site.
Observation pits will be dug at least 3 meters away from the transects to avoid disturbing the instrumentation. Soil profile descriptions will be made with the assistance of MLRA Soil Scientists. In addition, soil cores will be taken at each major horizon for lab determination of hydraulic conductivity (see subsequent section). In-situ hydraulic conductivity will be measured in triplicate in each major horizon. Procedures and data analysis will follow the standard methods (Amoozegar, 2004; Amoozegar and Wilson, 1999). Wastewater long term acceptance rate (LTAR) determinations will be made based on local rules and regulations.
Data Analysis
Hydrologic data will be analyzed to determine what parts of the study period fell within a wet, normal, or dry precipitation period. Correlation of redox features to saturation and reduction (as measured by redox probes) will be made. Hydraulic conductivity comparison between field methods and lab measurements will be made using standard ANOVA procedures. LTAR based on soil morphology will be compared to hydraulic conductivity measurements.
Data collected via the methods described previously will be used to model water table response to predicted changes in rainfall, temperature and other pertinent climatic conditions. Several hydrologic models will be used for comparison (e.g. Colorado State, DRAINMOD, topographic wetness index of SWAT model). Vepraskas et al. (2004) used DRAINMOD to correlate soil color to historic rainfall. Our approach will be to use various models to predict water tables based upon projected rainfall. This data can be used to compare current BMPs for system siting and design to determine if they will still allow for adequate separation distances to provide proper dispersal and wastewater treatment.
Additional Information
Incorporate additional criteria (such as soil features, physiography and hydrologic setting) that reflect site specific risks associated with potential climate change into the interpretation of site conditions or soils data used to determine wastewater hydraulic loading rates, soil wetness and seasonal high groundwater elevations which leads to almost certain system malfunction. Existing tools will be reviewed and new predictive tools (EM, VNIR for estimations of clay, silt, LIDAR for landscape interpretation) and models (fitting complex functions to VNIR estimates) must be researched and developed to provide site and soil evaluators with the best state-of-the-science methods and model functions. These tools must be developed to reflect the wide variations in soils, geology, climate, and hydrology found across the United States. We will investigate if evaluation methods and models used in other hydraulically-dependent land uses (e.g. storm water management, validation of CEAP watersheds etc.) can be applied to OWTS.
System Surveys
OWTS will be surveyed to assess system performance, evaluate what soil and site conditions are commonly related to failure and analyze system design and installation. Soil morphology, system installation depth, system installation procedures, system design and current functional status will be documented on each site. Local Health Department records will be searched for available information on original site evaluations, siting and installation specifications as well as troubleshooting activities related to malfunctioning systems.
Surveys will be conducted during the wettest period of the year, typically early spring or late fall (see Lindbo, et al., 1998). Field personnel will be trained in survey logistics and protocol, including interaction with the client. The team will document site details including system configuration/location relative to design and any encroachments and other cultural development. Nature and condition of vegetative cover and any associated water management strategies (diversion/drainage) will be described and noted. At least one soil boring will be made in original soil either between trenches or outside of the STA on each site. Data collected will include texture and structure as well as depth to redoximorphic features, saturation and perched water. A system will be considered malfunctioning if trenches exhibit pressure release upon probing or if effluent is surfacing.
Sites may also be evaluated using electromagnetic induction and related technologies to determine the parameters of the pollutant plume moving away from the system.
Objective 4. Develop educational materials and tools to acquaint the public and practitioners to management, operation, maintenance and health issues related to OWTS in light of adaptation to climate change.
Proper design, installation, operation and maintenance (O&M) and management of OWTS are critical for long-term treatment performance and to minimize system life-cycle costs. This is important for any size system, and especially critical for small community cluster systems that utilized advanced treatment technologies where wastewater reuse and recycling has the greatest application. Utilizing research knowledge gained from Objectives 1 through 3, we will:
Develop a technology matrix table that would assist wastewater professionals and local, county and state decision makers to determine what OWTS technologies are best suited for nutrient and pathogen sensitive watersheds and to address various on-lot site constraints such as shallow groundwater tables, shallow bedrock, slowly and rapidly permeable soils and size restricted lots.
Develop and/or improve outreach training materials related to soil and site suitability for OWTS that address issues of diverse conditions and expected climate change. Outreach materials may consist of look-up tables, decision-making trees/tables, nomographs, cumulative probability graphs and spreadsheet tools.
Work with our industry and regulatory partners to develop and improve materials for design, operation, maintenance, installation, economics, planning, management and analyzing and diagnosing malfunctions of conventional and alternative and innovative individual and small community cluster decentralized wastewater treatment systems as it relates to adaptation to and mitigation of climate change. Existing training manuals would be modified and new ones developed to reflect adaptation to climate change and this information would be disseminated as described below.
Using the education materials described above, we propose to train OWTS practitioners, decision makers and the public. Delivery of materials will be at outreach workshops conducted at the existing network of Land Grant onsite wastewater training centers/programs across the United States. In addition, training materials will also be delivered at state, regional and national professional conferences and trade shows. Delivery methods will include lectures, e-Learning, distance learning venues and hands-on field exercises, using fact sheets, demonstration systems and props, Power Point slides, videos and DVDs.
Measurement of Progress and Results
Outputs
- Refereed journal articles and publications for industry/association conference proceedings related to: <ul>the treatment performance of onsite wastewater treatment systems; <li>the microbial and biogeochemical mechanisms by which treatment is achieved; <li>use of advanced treatment technologies to mitigate climate change; <li>determining the site and soil suitability for onsite wastewater treatment systems, and the wastewater treatment capabilities of soils; <li>the influence of climate change on predicting site and soil suitability for onsite wastewater treatment systems; <li>the influence of climate change on treatment system performance; the impact of onsite wastewater systems on groundwater recharge, stream base flow, and water quality; <li>the installation and operation and maintenance requirements of onsite wastewater systems; <li>the use, costs, and management of innovative and alternative systems in rural communities; <li>the use of reclaimed water for sustainability of sensitive watersheds and rural communities; <li>and improving the planning and management of conventional and alternative and innovative (individual and small cluster) onsite wastewater treatment systems .</ul>
- A series of training workshops and associated educational materials to transfer emerging information to onsite wastewater practitioners at state, regional and national venues
Outcomes or Projected Impacts
- Increased knowledge and understanding among the members of the project technical committee, the OWTS industry and wastewater practitioners concerning siting, design, installation, plus operation and maintenance of conventional and advanced wastewater treatment technologies that meet the growing challenges posed by climate change and the need to protect human and environmental health. Utilizing the knowledge gained from this work will help develop a better collective understanding of new methods, processes, tools, findings and research questions yet to be solved.
- Using the knowledge gained from this research we will improve or develop new outreach demonstration education tools to help us communicate our research findings to our professional onsite wastewater practitioner communities. We expect information will be delivered at outreach workshops at existing onsite wastewater training centers and at newly developed centers, using fact sheets, DVDs, distance and e-Learning venues, and in lecture style venues at state, regional and at national conferences and trade shows. The new standards, methods, guidelines, protocols and training materials developed as part of this project will improve practitioner knowledge, encourage change in their attitudes and behavior, and foster systemic changes in regulations.
- We fully expect the knowledge base of onsite wastewater practitioners will continue to rise as the information developed from this project is delivered through outreach education and demonstration venues conducted under the auspices of Land Grant Institutions and state professional associations. The types and numbers of training classes where project research findings are delivered will be documented by the project technical committee members. To gauge the increased use of advanced treatment technologies we will work with state and county regulatory jurisdictions to determine the number of permit approvals for various advanced technologies. This will help us evaluate the effectiveness of our outreach activities related to new technologies.
- The breadth and depth of the above outcomes and projected impacts will reflect the level of funding from participating AESs and outside sources.
Milestones
(2011): Convene the first project meeting at NIFA National Water Conference. The purpose of this meeting will be to confirm leadership roles, assign duties to committee members to be completed during the tenure of the project and identify other scientists who might be interested in joining the committee. Report on progress, expand development of methods to address objectives and develop research-based outreach training materials and deliver materials at stakeholder training forums.(2012): Present and discuss progress made in meeting project objectives. Review, identify, improve and augment ongoing research methods based upon peer review and by utilizing evaluations, stakeholder feedback and comments, and experiences gained from training events. Develop, improve and enhance outreach education materials, deliver them at forums and assess the effectiveness of training efforts.
(2013): Review and assess progress made on research. Collect information to assess the numbers of practitioners being exposed to new research findings at training forums. Assess to what extent the practitioners being trained are utilizing the research-based training and associated technology they have received to change their behaviors or to effect change in their practices.
(2014): Present and discuss progress made in meeting project objectives. Identify, improve and augment ongoing research based upon peer review and by utilizing evaluations, stakeholder feedback and comments, and experiences gained from training events. Develop, improve and enhance outreach education materials, deliver them at forums and assess the effectiveness of training efforts.
(2015): Convene the final project meeting to discuss and evaluate prioritized research efforts, effectiveness of associated outreach forums. Present all completed standards of practice, developed publications and final committee reports.
Projected Participation
View Appendix E: ParticipationOutreach Plan
The results of this proposed multistate project will be published as project reports, on the project web site, as peer-reviewed publications, presentations at scientific meetings, presentations at industry conferences, and publications in industry magazines and newsletters. In addition, summaries of the completed work will be posted on participating members own web pages. Participating members involved in undergraduate teaching, graduate student advisement, and extension activities at Land Grant Universities will disseminate knowledge developed from the proposed project activities. Participants will disseminate information at local, regional and national stakeholder training workshops and classes that reach regulatory officials, professional engineers, land surveyors, environmental health specialist, soil scientists, registered sanitarians, OWTS installers, designers and maintenance service providers.
Organization/Governance
A regional technical committee will be organized upon project approval. Operational procedures to be followed will be according to those outlined in the NIFA Manual for Cooperative Regional Research. The voting members of the regional technical committee will include one representative from each cooperating agricultural experiment station or institution appointed by the director. The administrative advisor and the NIFA representative will be considered nonvoting members. All voting members of the technical committee will be eligible for office. The officers of the regional technical committee will be the chair, vice-chair, and secretary and will serve as the executive committee. These officers for the first year will be elected at the organizational meeting for the technical committee. In subsequent years, the officers will be elected annually and may succeed themselves. The chair, in consultation with the executive committee, will appoint subcommittees to facilitate the accomplishment of the various research and administrative tasks involving the cooperating institutional representatives. Such tasks may include, but are not limited to, research planning and coordination, development of specific cooperative research procedures, assimilation and analysis of data from contributing scientists, and publication of regional bulletins. The duties of the technical committee will be to coordinate work activities related to the project. The chair, in accord with the administrative advisor, will notify the technical committee of the time and place of meetings, prepare meeting agendas, and preside at meetings of the technical committee and the executive committee. The chair is responsible for preparing the annual progress report and coordinating the preparation of regional reports. The vice-chair assists the chair in all functions. The secretary records the minutes and performs other duties assigned by the technical committee or the administrative advisor. Annual meetings will be held by the technical committee for the purpose of conducting business related to the project. During each annual technical committee meeting, the subcommittees will report on their progress and identified needs to the entire committee. Considerable time will be devoted to the discussion of these reports.
Literature Cited
Amador, J. A., D. A. Potts, M. C. Savin, P. Tomlinson, J. H. Görres, and E. L. Nicosia. 2006. Mesocosm-scale evaluation of faunal and microbial communities of aerated and conventional septic system leachfield soils. Journal of Environmental Quality 35:1160-1169.
Amador, J.A., G.W. Loomis, D. Kalen, E.L. Patenaude, J.H. Gorres and D. A. Potts. 2007. Evaluation of leachfield aeration technology for improvement of water quality and hydraulic function in onsite wastewater treatment systems. Final Report to NOAA/UNH Cooperative Institute for Coastal and Estuarine Environmental Technology (CIDEET). Grant No. NA04NOS4190109. Univ. of New Hampshire. 27 pp.
APHA, 1998. Standard Methods for the Examination of Water and Wastewater. American Public Health Association, Washington, DC.
Amoozegar, A. 1988. Preparing soil cores collected by a sampling probe for laboratory analysis of soil hydraulic properties. Soil Sci. Soc. Am. J. 52:1814 1816.
Amoozegar, A. 2004. Soil permeability and dispersion analysis. p. 625-677. In R. D. Down and J. H. Lehr (ed.). Environmental Instrumentation and Analysis Handbook. John Wiley and Sons, Inc. Hoboken, New Jersey.
Amoozegar, A., and G. V. Wilson. 1999. Methods for measuring hydraulic conductivity and drainable porosity. p. 1149-1205. In R. W. Skaggs and J. van Schilfgaarde (ed.) Agricultural Drainage. Monograph No. 38, ASA-CSSA-SSSA, Madison, WI.
Bouchard, D. C., et al., 1992. Nitrate Contamination of Groundwater: Sources and Potential Health Effects, Journal of AWWA. 84(9):85-90.
Bouma, J. 1975. Unsaturated flow during soil treatment of septic tank effluent. J. Am. Soc. Civil Eng. 1:967-983.
Brown, D.F., L.A. Jones and L.S. Wood. 1994. A pedologic approach for siting wastewater. Proceedings of the Seventh National Symposium on Individual and Small Community Sewage Systems. American Society of Agricultural Engineers. pp. 229-237.
Burt, R. (ed). 2004. Soil Survey Laboratory Methods Manual. Soil Survey Investigations Report No. 42, Version 4.0. USDA-NRCS, National Soil Survey Center, Lincoln, NE.
Dane, J. H., and J.W. Hopmans. 2002. Water retention and storage. In: J. H. Dane, and G. C. Topp (eds). Methods of Soil Analysis Part 4: Physical Methods. No. 5 in Soil Sci. Soc. Am. Book Series. Soil Sci. Soc. Am., Madison, WI.
Emerick, R.W.,R.M. Test, G. Tchobanoglous and J. Darby. 1997. Shallow intermittent sand filtration: Microorganisms removal. Small Flows Journal 3(1):12-22.
Gold, A.J., W.R. DeRagon, W.M. Sullivan and J.L. LeMunyon. 1990. Nitrate nitrogen losses to groundwater from rural and suburban land uses. J. Soil and Water Conservation. 45(2):305 310.
Gold, A. J. and J. T. Sims. 2000. Research needs in decentralized wastewater treatment and management: A risk-based approach to nutrient contamination. National Research Needs Conference Proceedings: Risk-Based Decision Making for Onsite Wastewater Treatment, EPRI, US EPA, National Decentralized Water Resources Capacity Development Project. 2001.1001446, Palo Alto, CA.
Gold, A.J., B.E. Lamb, G.W. Loomis, J.R. Boyd, C.G. McKiel and V.J. Cabelli. 1992. Wastewater renovation in buried and recirculating sand filters. J. of Envir. Qual. 21:724 729.
Hallberg, G. R., 1989. Nitrate in Ground Water in the United States, in Nitrogen Management and Groundwater Protection, (Edited by R. F. Follett) Elsevier Sci. Publ., Amsterdam.
Hantzche, N. N. and E.J. Finnemore. 1992. Predicting groundwater nitrate-N impact. Ground Water. 304:490-499.
Hayhoe, K., C.P. Wake, T.G. Huntington, L. Luo, M.D. Schwartz, J. Sheffield, E.F. Wood, B. Anderson, J. Bradbury, A. DeGaetano, T. Troy and D. Wolfe. 2006. Past and future changes in climate and hydrological indicators in the U.S. Northeast. Climate Dynamics. DOI 10.1007/s00382-006-0187-8.
Holden, S.A., M.H. Stolt, G.W. Loomis and A.J. Gold. 2004. Seasonal variation in nitrogen leaching from shallow narrow drainfields. Proceedings of the Tenth National Symposium of Individual and Small Community Sewage Systems. American Society of Agricultural Engineers. pp. 432-440.
Horsley Witten Hegeman. 1991. Quantification and control of nitrogen inputs to Buttermilk Bay, Vol. 1. Barnstable, Massachusetts. 32 pp.
Kaplan, O.B. 1991. Septic systems handbook. Second edition. Lewis Publishers, Chelsea, MI.
Keeney, D.R. 1986. Sources of nitrate to ground water. CRC Critical Reviews in Environ. Control. 16:257-304.
Kraighera, B., T. Kosjek, E. Heath, B. Kompar and I. Mandic-Muleca. 2008. Influence of pharmaceutical residues on the structure of activated sludge bacterial communities in wastewater treatment bioreactors. Water Research 42: 4578-4588.
Lamb, B.E., A.J. Gold, G.W. Loomis and C.G. McKiel. 1990. N removal for on-site sewage disposal: A recirculating sandfilter/rock tank design. Trans. ASAE. 33(3):525-531.
Lamb, B.E., A.J. Gold, G.W. Loomis and C.G. McKiel. 1991. N removal for on-site sewage disposal: Field evaluation of a buried sand filter /rock tank design. Trans. ASAE. 34 (3):883-889.
Lindbo, D. L., T. M. Campbell, N. Deal, and R. Hollowell. 1998. Performance of sand lined trench and conventional systems within a management entity. Proceedings of the Eighth National Symposium on Individual and Small Community Sewage Systems. American Society of Agricultural and Biological Engineers. pp. 177-185.
Lindbo, D.L., J. Lynn, K. Neal, G. Young and A. Amoozegar. 2007. Applying soil morphology to long term acceptance rate determination. Proceedings of the Eleventh National Symposium on Individual and Small Community Sewage Systems. American Society of Agricultural and Biological Engineers. 10pp.
Loomis, G.W., D.B. Dow, M.H. Stolt, L.T. Green and A.J. Gold. 2001. Evaluation of innovative onsite wastewater treatment systems in the Green Hill Pond Watershed, Rhode Island. Proceedings of the Ninth National Symposium on Individual and Small Community Sewage Systems. American Society of Agricultural Engineers. pp. 505-514.
Loomis, G., D. Dow, J. Jobin, L. Green, E. Herron, A. Gold, M. Stolt, and G. Blazejewski. 2004. Long-term treatment performance of innovative systems. Proceedings of the Tenth National Symposium of Individual and Small Community Sewage Systems. American Society of Agricultural Engineers. pp. 208-218.
McCray, J. et al. 2010. Quantitative tools to determine the expected performance of wastewater soil treatment units: Toolkit users guide. Water Environmental Research Foundation. http://www.werf.org//AM/Template.cfm?Section=Home.
Miles, R.J., and L.T. West. 2001. Soil-based assessment of site suitability for on-site wastewater treatment systems: a comparison of Georgia and Missouri systems. Proceedings of the Ninth National Symposium on Individual and Small Community Sewage Systems. American Society of Agricultural Engineers. pp. 62-70.
Morgan, C.P.. 2002. An investigation of soil morphology-water table relationships on Block Island. Masters Thesis. University of Rhode Island. Kingston, RI.
Morgan, C.P. and M.H.Stolt. 2006. Soil morphology-water table cumulative duration relationships in southern New England. Soil Science Society of America Journal 70: 816-824.
Obropta, C.C., and D. Berry. 2005. Onsite Wastewater Treatment Systems: Alternative Technologies. Rutgers University Fact Sheet. (http://www.water.rutgers.edu/Fact_Sheets/fs530.pdf)
Patz, J.A. et al. 2005. Impact of regional climate change on human health. Nature. 438:310-317.
Postma, F.B., A.J. Gold and G.W. Loomis. 1992. Nutrient and microbial movement from seasonally-used septic systems. J. Environ. Health 55:5-10.
Potts, D. A., J. H. Gorres, E. L. Nicosia, and J. A. Amador. 2004. Effects of aeration on water quality from septic system leachfields. Journal of Environmental Quality 33:1828-1838.
Rich, B., 2005. Experiences with denitrifying onsite systems in the La Pine area of Central Oregon. Proceedings of the Thirteenth Northwest On-Site Wastewater Treatment Short Course. University of Washington, Seattle. (http://www.engr.washington.edu/epp/wwt/proceedings/)
RIDEM. 2008a. Innovative and alternative system use in Rhode Island. Personal communication with Brian Moore, Supervising Sanitary Engineer, Rhode Island Dept. of Environmental Management. Providence, RI.
RIDEM. 2008b. Rules Establishing Minimum Standards Relating to Location, Design, Construction and Maintenance of Onsite Wastewater Treatment Systems (January 1, 2008). Rhode Island Dept. of Environmental Management. Providence, RI.
Ritter, W.F. 1986. Nutrient budgets to the inland bays. Technical report. Delaware Department of Natural Resources and Environmental Control. Dover, DE. 70 pp.
Robertson, W. D., S. L. Schiff and C. J. Ptacek. 1998. Review of phosphate mobility and persistence in 10 septic system plumes. Ground Water 33(6): 1000 -1010.
Ryther, J. H. and W. M. Dunstan. 1971. Nitrogen, phosphorus and eutrophication in the coastal marine environment. Science 171: 1008-1013.
Scandura, J. E. and M. D. Sobsey. 1997. Viral and bacterial contamination of groundwater from on-site sewage treatment systems. Water Science and Technology 35(11-12): 141-146.
Schaub, S. A. and C. A. Sorber. 1977. Virus and bacteria removal from wastewater by rapid infiltration through soil. Applied Environmental Microbiology 33(3): 609-619.
Siegrist, R.L. and P.D. Jenssen. 1989. N removal efficiency during wastewater infiltration as affected by design and environmental factors. Proceedings Sixth Northwest On-Site Wastewater Treatment Short Course. University of Washington. Seattle. pp. 304-318.
`imonek, J., M.Th. van Genuchten, and M. `ejna. 2006. The HYDRUS Software Package for Simulating Two- and Three-Dimensional Movement of Water, Heat, and Multiple Solutes in Variably-Saturated Media, Technical Manual, Version 1.0, PC Progress, Prague, Czech Republic, 241.
Stolt, M.H., B.C. Lesinski and W. Wright. 2001. Micromorphology of seasonally saturated soils in carboniferous glacial till. Soil Science 166: 406 414.
Tomaras, J., J. W. Sahl, R. L. Siegrist, and J. R. Spear. 2009. Microbial diversity of septic tank effluent and a soil biomat. Applied and Environmental Microbiology 75:3348-3351.
Tyler, E.J., E. M. Drozd, and J.O. Peterson. 1991. Estimating wastewater loading rates using soil morphological descriptions. Proceedings of the Sixth National Symposium of Individual and Small Community Sewage Systems. American Society of Agricultural Engineers. pp. 192-200.
Tyler, E.J. and J.C. Converse. 1994. Soil acceptance of onsite wastewater as affected by soil morphology and wastewater quality. Proceedings of the Seventh National Symposium of Individual and Small Community Sewage Systems. American Society of Agricultural Engineers. pp. 185-194.
Tyler, E.J. 2001. Hydraulic wastewater loading rates in soil. Proceedings of the Ninth National Symposium of Individual and Small Community Sewage Systems. American Society of Agricultural Engineers. pp. 80-86.
USEPA. 1983. Final environmental impact statement: Wastewater management in rural lake areas. U.S. Environmental Protection Agency Region 5, Water Division. Chicago. 287pp.
USEPA. 1997. Response to Congress on Use of Decentralized Wastewater Treatment Systems. USEPA Office of Water. EPA 832-R-970-001b.
USEPA. 2002. Onsite Wastewater Treatment Systems Manual. U.S. Environmental Protection Agency, Office of Water. EPA /625/R-00/008. Washington, DC.
Vaughn, J. M., E. F. Landry and M. Z. Thomas. 1983. Entrainment of viruses from septic tank leach fields through a shallow, sandy soil aquifer. Applied Environmental Microbiology 45(5): 1474-1480.
Vepraskas, M. J., X. Hea, D. L. Lindbo and R. W. Skaggs. 2004. Calibrating Hydric soil field indicators to long-term wetland hydrology. Soil Sci. Soc. Am. J. 68:1461-1469
Weiskel, P. K. and B. L. Howes. 1992. Differential transport of sewage-derived N and phosphorus through a coastal watershed. Environmental Science and Technology 26(2): 352-360.
Whitmeyer, R. W., R.A. Apfel, R.J. Otis and R.L. Meyer. 1991. Overview of individual onsite nitrogen removal systems. Proceedings of the Sixth National Symposium of Individual and Small Community Sewage Systems. American Society of Agricultural Engineers. pp. 143-154.