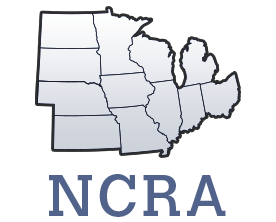
NC_OLD1178: Impacts of Crop Residue Removal for Biofuel on Soils (formerly NC1017)
(Multistate Research Project)
Status: Inactive/Terminating
NC_OLD1178: Impacts of Crop Residue Removal for Biofuel on Soils (formerly NC1017)
Duration: 10/01/2009 to 09/30/2014
Administrative Advisor(s):
NIFA Reps:
Non-Technical Summary
Statement of Issues and Justification
The growing importance of renewable energy, specifically as biofuels, is indicative of the insatiable energy demands of the globalizing and growing world economy but hidden in this is the high economic and environmental costs of fossil fuels. In his State-of-the-Union address on 23 Jan. 2007, President Bush enthusiastically proposed to cut U.S. gasoline consumption by up to 20% by 2017 through an increase in ethanol production to 35 billion gallons a year, or a fivefold increase over current ethanol production requirements. There are now 113 North American ethanol plants, with an additional 77 under construction, primarily sited in the Corn Belt of the Midwest. Most of the existing ethanol plants are corn grain-to-ethanol refineries. The long-term goal is to produce 60 billion gallons of ethanol per year to replace 30% of the ground transportation fuel demand of the U.S. To meet these demands, the use of crop residues is being considered as a source of lignocellulosic feedstock for producing ethanol (Wilhelm et al., 2004), although it is not yet a routine practice (Johnson et al., 2004). One ton of corn stover or any other cellulosic biomass could theoretically produce 100 gallons of ethanol. An annual production of 60 billion gallons of ethanol from cellulosic biomass would require 600 million tons of biomass per year. The overall goal for ethanol production is to procure 1 billion tons of biomass for the U.S. (Somerville, 2006) and about 4 billion tons for the world. The current rate of residue production from all crops is about 500 million tons in the U.S. and about 4 billion tons in the world. Some analysts argue that 300 million tons of corn residue could be harvested for meeting the U.S. ethanol demands. Similar to the U.S., 34 straw-burning power plants were being built at the end of 2006 in rural areas in China with a capacity of producing 1.2 million kW of power. The straw-burning power industry is likely to grow in China and associated geographic regions. Of the 600 million tons of straw produced annually, China plans to use 300 million tons to produce energy. India, which produces about 440 million tons of crop residues annually, also has plans to build straw-burning power plants. When crop residue is removed from soil there is increased potential for soil degradation, and erosion resulting in a subsequent decline in soil quality, reduction in crop yield, and decrease in local and regional water quality (Simpson et al., 2008). Crosson (1984) estimated a monetary loss in the U.S. from soil erosion due to reduced cropland productivity to be 40 million dollars in a given year. In 2001, den Biggelaar et al. (2001) estimated the loss at $55.6 million. Others, Crosson (1986) and Pimentel et al. (1995), have estimated the loss to be over $100 million. The results vary depending on the erosion rates, weather, and crop prices for the years covered in the study. For many soils, continued erosion results in degraded and reduced topsoil thickness. Reduced crop yields occur as root restrictive layers, such as fragipans, subsoil horizons with a large amount of clay, or coarse sand become closer to the soil surface (Langdale et al., 1985). Offsite damages to the environment caused by soil erosion and subsequent deposition of sediments in the U.S. are considerable (Pimentel, 1992). Deposition of eroded soil materials in surface water bodies such as reservoirs, lakes, rivers, and streams cause a decline in water quality, reduce environmental quality, and decrease the functional life expectancy of reservoirs. Eroded sediments often contain not only soil materials from the organic surface soil which are enriched in nutrients and C, but often include commercial and/or organic (animal waste) fertilizers, pesticides, and agricultural pharmaceuticals (from animal waste). Excessive removal of crop residues, particularly corn stover, can adversely affect soil quality and exacerbate the problem of soil erosion. Residue removal increases the susceptibility of the soil surface to crusting/increased surface sealing because of rainfall-induced consolidation and abrupt wetting and drying (Or and Ghezzehai, 2002; Blanco-Canqui et al., 2006). Residue mulch increases aggregation (Mochizuki et al., 2008), improves soil hydraulic properties (Strudley et al.2008; Blanco-Canqui et al., 2007) and intercepts raindrops responsible for crust-forming processes such as detachment of soil particles and dispersion of surface aggregates. Crusts are thin soil surface layers about 5 cm thick (USDA-NRCS, 1996), but they are denser and less permeable than the underlying soil layers (Busscher and Bauer, 2003). Because of their greater strength and low permeability, crusts can modify soil surface processes, restricting seedling emergence (Baumhardt et al., 2004), reducing water infiltration and aeration (Wells et al., 2003), and increasing surface runoff (Bajracharya and Lal, 1998) thus inducing a greater level of soil erosion with long-term detrimental effects on plant growth (Maiorana et al., 2001). Stover mulch also reduces the abrupt fluctuations in soil water regimes (Black 1973a). Soils with stover mulch often have greater water content that those without mulch (Shaver et al., 2002). Soil water content is the single most important factor essential to plant growth, evapotranspiration, biological activity, nutrient movement, and other vital soil processes. Some researchers have estimated that ranges of about 30% (Nelson, 2002), 40% (Kim and Dale, 2004), and 58% (Lindstrom et al., 1979) of the total corn stover production in the U.S. Corn Belt region may be available for biofuel production. These removals are, however, based mainly on the residue requirements to reduce soil erosion risks and not on the needs to moderate soil surface strength or soil C sequestration. Allowable removal rates of corn stover based on the need to reduce soil erosion in the U.S. Corn Belt region are site-specific (Lindstrom et al., 1979; Nelson, 2002; Kim and Dale, 2004). Thus, the quantity of stover that must be retained on the soil to reduce crusting is also likely to depend on site-specific conditions such as tillage and cropping system (Kladivko, 1994), duration and intensity of management (Karlen et al., 1994), soil properties (Gupta et al., 1987), as well as agro-ecosystem and climate (Salinas-Garcia et al., 2001). Knowledge of the threshold levels of stover removal in relation to soil crust strength and water storage is urgently needed to design residue management options for biofuel production while maintaining soil physical quality, reducing risks of pollution of surface water, and sustaining agricultural productivity. While studies on the interacting effects of traditional tillage systems vs. residue management on soil strength properties are many (Larson et al., 1978; Lindstrom et al., 1979; Kladivko, 1994), changes in crust strength parameters and water content regimes resulting from differential corn stover retention in no-tillage (NT) systems are not well documented (Blanco-Canqui et al., 2006). Moreover, the magnitude of the impacts of crop residue removal on soil crust strength properties can be variable depending on soil textural characteristics and management (Morachan et al., 1972; Black, 1973b; Gupta et al., 1987; Karlen et al., 1994; Shaver et al., 2002). Many of the soils of the North Central region possess silty surface textures which are naturally more susceptible to crusting and physical degradation. Thierfelder et al. (2005) showed that cone index (CI) of crusted soils without vegetative cover was seven times greater than that of those with vegetative cover. In contrast, Karlen et al. (1994) observed that differences in CI and Áb of soils within the surface 5-cm depth after 10 yr of complete removal and double addition of stover mulch annually under NT continuous corn were not significant in Rozetta and Palsgrove silt loams. Decline in soil organic carbon (SOC) concentration and deterioration of soil structural properties as a result of stover removal can be significant. Several studies in the Midwestern U.S. Corn Belt region including those from Iowa (Larson et al., 1972), Indiana (Barber, 1979), Minnesota (Allmaras et al., 2004; Wilts et al., 2004), Wisconsin (Karlen et al., 1994); and Ohio (Blanco-Canqui and Lal, 2007a, b; Blanco-Canqui et al., 2007) have shown that stover removal reduces the SOC concentration. In other regions, reductions in SOC concentration due to stover removal may, however, be small or not significant (Hooker et al., 2005). The SOC concentration is also positively correlated with aggregate stability (McVay et al., 2006). Previous work by members of NC-1017 has demonstrated the importance of storing soil C (through increasing organic matter) for improving soil quality, including soil physical properties such as improved water holding capacity (Lal, 1999). However, a strong connection between soil erosion and the global C balance has not been well established. There is also a need for developing sound methodology for obtaining a quantitative estimate of the actual distribution of soil C on various eroded and noneroded landscapes in the Midwest. It was recognized during the Kyoto Protocol that net emissions of greenhouse gases, such as CO2 and CH4 could be decreased by either reducing emissions or by increasing the rate of C sequestration in soils. Agricultural soils are one of the largest reservoirs of C, and thus have a great potential to mitigate the increasing concentration of CO2 in the atmosphere (FAO, 2001). Evaluation of the C pool in soils is difficult because of its heterogeneity in time and space (FAO, 2001). The global loss of C because of erosion is estimated to be in the range of 150 to 1500 million tons per year (Lal, 1995; Gregorich et al., 1998; Lal, 2003) but the processes are not well understood. Erosion is a selective process involving detachment and transport of the light soil fraction consisting of soil organic carbon (SOC) and clay (Sharpley, 1985). The fate of eroded soil particles is complex depending on many parameters including soil properties, landscape elements and properties, drainage net and soil management. Many of the soil particles eroded are moved downslope and may remain in the same field or watershed for a considerable length of time. However, this movement results in increased spatial variability of soil properties across the landscape, especially soil organic matter and those elements of environmental concern that are associated with it - carbon and nitrogen (Schumacher et al., 1999). This proposal outlines a project designed to help better understand changes in soil quality including soil degradation resulting from crop residue removal for biofuel production and animal production systems. It will also provide needed data on the changes in the soil C reservoir related to intensive land use and residue removal for some of the major soils in the North Central region. This study will contribute to our understanding of soil-landscape processes with the potential to provide data that will contribute to improved management of our soil and water resources. We view this approach as a natural progression related to the past research efforts of NC-174 and NC-1017. Knowledge gained from the proposed research will contribute to a more quantitative understanding of the effects of agroecosystem management (crop residue removal) on global C balance; erosional processes; the amounts and landscape distribution of C and organic matter; and changes in soil quality. The proposed regional research project will provide information that can be used to enable sustainable management of natural resources in different ecosystems, over the varying climates and soil landscapes that occur in the participating states. The North Central Regional Association of Agricultural Experiment Stations has a current list of research priorities for seven cross-cutting research areas and objectives. The proposed Regional Project addresses four of these objectives for the Natural Resources and the Environment. These include: (1) Understand the ecological processes operating in human, plant and animal communities, (2) Define sustainable principles for resource management, utilization and land use, (3) Identify and apply ecosystem management principles and practices for the utilization and protection of resources, restoration of natural systems and management of rural landscapes, and (4) Assess the relationships of agricultural/forestry practices (primary production) upon soil and water systems and bio-diversity. Our project will contribute to these research priorities.
Related, Current and Previous Work
In addition to erosion and residue removal, SOC concentration is influenced by management practices. There have been many studies on residue management for diverse tillage and cropping systems (e.g., Lal et al., 1980; Dabney et al., 2004; Sharratt, 1996; Singh et al., 1996; Karlen et al., 1994; Findeling et al., 2003). Formation and preservation of earthworm macropores in NT systems are well known (Bohlen et al., 1997; Butt et al., 1997; Shipitalo and Butt, 1999). Since stover left on the soil surface provides an abundant food source and habitat to earthworms responsible for macropore network development, its removal from NT systems may reduce earthworm populations and the number of surface-connected macropores. Earthworm burrowing and feeding activities enhance soil structure and macropore development, recycle essential nutrients, and control rates of SOC turnover and water-air flow in the soil profiles (Bohlen et al., 1997). Surface sealing of open and continuous macropores due to stover removal can be a major factor in reducing near-surface parameters of water flow and gaseous diffusion and transport such as hydraulic conductivity of saturated soil (Ksat), air permeability (Ka; Ela et al., 1992; Loll et al., 1999). The Ka and Ksat are functions of macropore structure and are key indicators of soil structural development (Loll et al., 1999). Changes in earthworm populations and soil macroporosity and their relations to Ksat, Ka and soil water retention (SWR) resulting from systematic stover removal are poorly understood. Effects of removing wheat (Triticum aestivum L.) and soybean (Glycine max L.) residues have been investigated (Pikul and Allmaras, 1986; Skidmore et al., 1986), but few studies have assessed corn stover removal impacts on soil hydraulic properties (Karlen et al., 1994). Corn stover removal may affect soil properties differently from that of wheat and soybean residues because corn stover is coarser, less readily decomposable, and thus remains longer on the soil surface (Mankin et al., 1996). Erosion can impact the soil as a source or sink of CO2, CH4, and other greenhouse gases, depending on soil quality and the predominant pedospheric processes (Bouwman, 1990; Lal et al., 1995a; b; Lal, 2003). Whereas some of the C transported into the aquatic ecosystems and depressional sites may be buried and sequestered, as much as 15 Tg C/y in the U.S. (Lal, 2003) and 1 PgC/y in the world (Lal, 2003) may be emitted into the atmosphere because of erosional processes. Adopting conservation-effective measures for erosion control can minimize risks of erosion-induced emissions. Further, increases in SOC and soil inorganic C (SIC) contents, by using best management practices (BMPs) and soil restorative measures, can make landscape and soil a net sink for atmospheric C and reduce or mitigate the greenhouse effect (FAO, 2001). Decreases in SOC and SIC contents, because of accelerated soil erosion and attendant soil degradation leading to decline in biomass productivity, can exacerbate the greenhouse effect due to emissions of CO2 and CH4 from soil to the atmosphere. Soil erosion leads to on-site depletion of SOC content because of transport of dissolved organic C (DOC) and particulate organic carbon (POC) in runoff and eroded sediments to their receiving environments. Accelerated erosion also enhances the rate of mineralization by breaking down aggregates and exposing the hitherto protected or encapsulated carbon to microbial processes and enzymatic action; thus, an increase of C gases to the atmosphere. Bajracharya et al. (2000a;b) reported significant variations in CO2 flux among seasons in Ohio but found no direct effect of erosion phase on C flux from the soil. In contrast, Parkin and Kaspar (2003) reported that an eroded Clarion soil (Typic Hapludoll) had greater CO2 flux than an adjacent slightly eroded Endoaquoll. Similarly, the same investigators (Parkin and Kaspar, 2003) reported greater respiration/flux on eroded sites and diurnal patterns of CO2 flux. Previous Accomplishments Past and future NC-1017/NC-TEMP-1017/NC-174/NCT-199 research efforts are summarized in Table 1 (see attached). The sequence represents a natural progression from studying soil productivity-erosion relationships to determining C distributions, dynamics and sequestration in eroded landscapes. During the first 5-year phase of the NC-174 project (1983-1988) we identified and documented the effects of erosion on soil properties and corn or small grain yield for research sites located in 11 states (Table 1). Five years of data were collected to better document the effects of weather on the interaction between soil properties and corn yield in the north central United States. First phase achievements included 13 refereed journal articles and chapters in books. Once the database was enlarged, emphasis was placed on selection of management and restoration alternatives at either the initial or a new research site (Table 1, phase 2). The NTRM (Nitrogen, Tillage, Residue and Management) and EPIC (Erosion-Productivity Impact Calculator) models were used in conjunction with the existing data base collected during the initial phase of the NC-174 project to identify factors limiting crop productivity of each soil series investigated. The models were used to evaluate long-term effect of management and restoration alternatives prior to field testing. The second phase (Table 1) of the project (1988-1993) was to field test the practices selected to maintain or enhance current productivity and to determine the extent to which productivity of eroded soils can be restored. Phase 2 outputs included 25 journal articles and chapters in books. The third phase (Table 1) of the project (1993-1998) determined threshold soil property values for the restoration of productivity and quality of eroded soils to initial levels. Third phase accomplishments included 74 refereed journal articles and chapters in books. During the fourth phase (Table 1) of the project, from 1998 to 2003, we examined the erosional and landscape impacts on soil processes and properties as well as assessed the management effects on eroded soil productivity and the quality of soil, air, and water resources. Achievements during phase 4 of the project included 70 refereed journal articles and chapters in books. In the fifth phase (2004-2009) (Table 1) the main focus was on C distribution and sequestration within the landscape. This focus was integrated with the previous phase by examining the impact of management and erosion on C distribution within the landscape and related to soil quality and productivity. Fifth phase achievements included 84 refereed journal articles and chapters in books. The fifth phase included representation external to the North Central region including participants from Guam and Manitoba. The sixth phase of this study (Table 1) will build on previous achievements by examining the role of crop residue removal on factors affecting crop productivity and carbon sequestration. The findings from these studies will be linked to existing studies that relate management and erosion on C distribution within the landscape to soil properties associated with soil quality and productivity. Related Work There are two multistate projects that are related to our proposed project. These include NC-1032 (Characterizing active soil organic matter pools controlling soil N availability in maize-based cropping systems) and NCERA-59 (Soil organic matter; formation, function, and management). NC-1032 objectives primarily relate to N management and its effect on soil organic matter pools. The objectives of NC-1032 and NC-TEMP-1017 are complimentary with no substantial overlap. NC-1032 objectives include: 1. Conduct fundamental work to enhance current understanding of the role of active N and C pools in cropping systems and to predict N mineralization toward more efficient use of N fertilizers. 2. Assess the response of crop N uptake to varying rates of N fertilizer in on-farm trials and explain the responses through the dynamics of the quantitites and chemical properties of active fractions of soil C and N, including the light fraction and the mobile humic fraction. 3. Quantify the magnitude and annual variation in the indigenous soil N supply in on-farm trials as influenced by key edaphic properties, climate, and cropping system. NCERA-59 is an unfunded committee that does not coordinate joint research across participants. The objectives of NCERA-59 are broad and diverse. These include i. promoting information exchange on soil organic matter; ii. identifying and evaluating soil indicators for soil ecological management; iii. conducting outreach activities to promote the ecological management of soils; iv. co-sponsoring symposiums on soil organic matter and soil quality; and v. interacting with other regional committees. NC-TEMP-1017 currently has one individual who is also a member of NCERA-59. The two multistate committees have been in communication with each other and have jointly participated in combined meetings in the past. The findings from the NC-TEMP-1017 project also have the potential of adding synergistically to the activities of three other multi-state committees. These include NCERA-180 (Site-specific crop management); SERA038 (Biobased energy research and information exchange committee); and NC-506 (Sustainable biorefining systems for corn in the North Central Region). Our emphasis on measurements within landscape positions in eroded fields will provide information that can be used in the development and improvement of site-specific crop management in marginal lands used for biofuel production systems. Additionally the output from our committee will integrate well with the objectives of multi-state committees who are broadly examining biofuel production strategies. There is minimal overlap of other CRIS projects with NC-TEMP-1017/(NC-1017) which has focused more on the chemical and physical properties of soil with the biological component limited primarily to total C or total N. The new proposed NC-TEMP-1017/(NC-1017) project emphasis relates to the effects of management and residue removal on soil organic carbon and its interaction with soil quality with an emphasis on soil surface properties such as crusting and infiltration. An important focus of this proposal is the examination of spatial and temporal changes in soil properties within eroded and eroding landscapes associated with different soil management systems that are being developed for biofuel production. Assessment of active C pool levels within the soil landscapes is critical to understanding the dynamics of C levels on productivity and soil physical properties. Surface soil properties are most likely to be the first indicators of a change in soil quality moderated by residue removal and soil carbon loss. Additionally land that has undergone significant past erosion may be the most sensitive to residue management strategies. A search of CRIS projects associated with the combined terms of soil organic carbon, residue, and erosion resulted in an output of two projects. Both projects terminated in 2006. One project was designed to quantify basic nutrient transformation and transport processes, while the second project emphasized the measurement of greenhouse gases in corn-soybean rotations. Our proposal is unique among multistate projects and individual CRIS projects in that we address the issues of soil carbon-soil quality interactions in an integrated approach within eroded landscapes that are likely to be managed for biofuel production.
Objectives
-
Assess management effects on carbon sequestration and soil productivity including the impacts of crop residue removal on soil organic carbon (SOC) and erosion.
-
Determine spatial C distribution and dynamics in soils of eroded landscapes for better quantification of erosion impacts on soil carbon loss and sequestration.
Methods
Objective 1. Assess management effects on carbon sequestration and soil productivity including the impacts of crop residue removal on soil organic carbon (SOC) and erosion. All states will participate in Objective 1. A model will be used that includes the elements of a hillslope. In each participating state, a minimum of two sites with contrasting ecosystems but similar soil-landscape relationships will be sampled. For most states the least disturbed ecosystem will be native grass or timber, depending on the location in the NC and other Multi-State regions. The other site will be an intensively managed agroecosystem used for continuous row crop or wheat production. Different rates of crop residue management, to be implemented on replicated plots, will include selected rates of removal and addition of crop residue from the previous year. The percentage of stover mulch cover in each plot will be estimated using the line transect or photograph method or equivalent methods (Sloneker and Moldenhauer, 1977; Lowery et al, 1984). All treatments will be established, if possible, in a randomized completed block design, with a minimum of three plots per treatment. Initial soil carbon data will be collected prior to treatment establishment. Three soil cores should be collected from center of each hillslope element (treatment) with a 120-cm long, 6-cm diameter solid steel sampling tube containing a 5.7-cm acetate contamination liner to a recommended minimum depth of 1 meter (sampling depth may depend on thickness of soil root zone and presence or absence of carbonates). In the laboratory, the cores will be cut into 5-cm, 15-cm, or 30-cm sections and core bulk density will be determined on each section. Each core section will constitute one soil sample. The soils will be air-dried and crushed to pass a 2-mm screen. The soil profiles will be described using standard procedures and sampled as described above. Particle-size analysis, pH, bulk density, total C and SIC (if carbonates are present) or SOC (if carbonates are not present), and moisture at time of sampling will be completed for each sample. A minimum of three cores will be collected per treatment to compensate for soil variability within the local study area. No less than two cores will be collected per treatment to account for variability. Preference will be given to section the cores by defined depth increments rather than by horizon because it is simpler to later compile the data to calculate C mass in the soil. Horizons are not the same thickness from plot to plot or treatment-to-treatment. The depth increments will not be less than 5-cm and will be selected based on the soils and treatments being studied. Ideally, the first one or two increments from the surface should be 5-cm because of the expected rapidly changing differences due to management practices. Deeper in the profile, the increments will be at least 15-cm. All the cores collected from a given study will have the same depth increments for ease of comparing treatment effects. Approximately 10-15 g subsamples will be further ground to pass a 100 mesh screen for C analysis. Total C analysis will be performed by high temperature combustion. If soil carbonates are present SIC will be determined by an accepted method, e.g. titrimetric, volumetric or pressure transducer. Quantities of SIC will be subtracted from the total C values to obtain SOC values. If soil carbonates are absent SOC will be determined by an accepted method (e.g., Nelson and Sommers, 1982). The C content of soils will be adjusted for soil bulk density and reported as kg C /m3 or Mg C/ha/m if sampled to 1 meter and as kg C /m2/ root zone depth or Mg C/ha/root zone depth if carbonates are present. A procedure manual will be developed to accommodate regional soil (such as presence or absence of carbonates) and weather differences to insure as much uniformity in sampling procedure and laboratory analyses as possible. The impact of crop residue removal on soil surface physical properties (including surface crusting-as indicated by cone index, infiltration, Ka, and Ksat) will be evaluated for the control (no residue removed or added under no-tillage system) and selected levels of residue cover of crop residue from the previous year. The surface Ka will be measured with an air permeameter (Ball and Schjonning, 2002; Grover, 1955). Surface soil Ksat will be measured with a tension infiltrometer (Clothier and Scotter, 2002; Wooding, 1968) in addition to infiltration measured with a simulated rainfall infiltrometer. Surface crust, as measured by cone index, will be measured with a cone penetrometer (Lowery and Morrison, 2002). A minimum of three of each of these measurements (cone index, infiltration, Ka, and Ksat) will be made in each plot. Data from physical property measurements will be analyzed with appropriate statistical method(s). Objective 2. Determine spatial C distribution and dynamics in soils of eroded landscapes for better quantification of erosion impacts on soil carbon loss and sequestration. This is a continuation of research from phase five. To assess soil C distributions we have collected all the C data and much of the necessary landscape data to construct multi-dimensional models for the research sites using a technique developed by Grunwald et al. (2001). This method requires soil profile penetration data for several soil horizons. Rooney and Lowery (2000) developed a profile cone penetrometer (PCP) for collecting such data. The PCP consists of a 30 degree cone with a 2.0 cm base diameter, threaded to a 1.25 cm in diameter by 1.5 m long stainless steel rod (ASAE, 2000). Penetration force is measured with a 1360-kg load cell, while depth will be measured using a string potentiometer. The PCP is pushed into the soil profile at a rate. An electronic data logger is used to collect load cell and string potentiometer data. A digital elevation model (DEM) is created from data collected with a differentially corrected global positioning system (GPS) unit attached to an all-terrain vehicle. Profile cone penetrometer sampling points will be geo-referenced with a GPS unit. Penetration force data will be transformed into cone index (CI) using CI = Fp / Ac, where Fp is the penetration force, and Ac is the basal area of the cone. At each recorded depth, there will be an associated CI value, thus creating a continuous curve for the entire profile at each sampling point. These data will then be analyzed using the Cluster Observation procedure in Minitab (Minitab, 2000), with the standardized variables option selected, and using the Squared Pearson and Ward method for distance measure and linkage method, respectively. The cluster procedure creates clusters, or groups, of observations that are similar. Thus, if soil erosion levels are desired, erosion levels will be delineated as a unique group of CI values. Profile CI values can then be clustered into three clusters which represent slight, moderate, and severe erosion levels. These data combined with GPS data and integrated with the output of soil erosion models can be used to create a soil maps displaying soil variability as a function of soil erosion and soil C data can be added. Soil erosion models (TEP and WATEM) (Lindstrom et al., 2000) are being used to estimate changes in the pattern of soil and C translocation in study sites. DEM developed by survey grade differential GPS data as described previously is being used as primary input into the models. Additional inputs will include rainfall erosivity (R), soil erodibility (K), and crop management factors (C)determined from local NRCS data. Soil bulk density is available from core samples previously collected under phase five and or objective 1. Tillage translocation coefficients (k) are assigned based on past tillage practices at the study sites. Patterns of soil translocation are evaluated using current erosion models. The resulting pattern will be compared with the measured distribution of C and profile cone penetrometer soil variability within the field (Schumacher et al., 2003, Grunwald et al., 2001). The spatial values and erosion estimates will be compared with soil C data to obtain a multi-dimensional soil carbon variability map. The contributions of water and tillage erosion in C movement within the study landscape will be estimated and compared with the pattern of C in the non-cultivated landscape (Schumacher et al., 2003).Measurement of Progress and Results
Outputs
- 1) Enhanced understanding of soil-landscape processes thus contributing to improved management of soil and water resources.
- 2) Documentation of changes in the soil C reservoir and surface soil physical properties related to residue management for selected major soils in the NC and other participating regions.
- 3) Contributions of information for use in decision aides designed for evaluation of biofuel production systems applied to marginal lands.
- 4) Analysis of the effects of erosion on the distribution of soil physical properties and soil carbon within undulating landscapes.
- 5) Scientific publications, guidebooks, and fact sheets that address the specific benefits of crop residue management practices on soil and crop productivity as well as environmental quality.
- Output 6 Website that provides documentation of research activities for the general public and a central coordinating site for committee collaboration.; Output 7 Organization of workshops and meetings designed to extend information to land managers and policy makers.
Outcomes or Projected Impacts
- 1) Reduced soil degradation in previously eroded landscapes. Information generated in this project will provide an analysis of the interaction of crop residue management impacts on soil organic carbon and soil surface properties critical to sustained biofuel and crop production applicable to a wide geographic region. We will have a better understanding of changes in surface soil properties resulting from intensive cropping and residue removal for biofuel production on soil quality among selected agroecosystems.
- 2) An increase in scientific knowledge concerning soil-landscape processes. Knowledge generated in this project will be useful for documenting economic and environmental benefits for adoption of conservation based biofuel production systems that enhance soil organic carbon and soil surface properties that sustain crop productivity in eroded landscapes. This information will foster improved management of our resources and enhanced environmental quality.
- 3) Biofuel and conservation policies and management practices based on science based information. Information generated from this project will provide information based on regional coordinated experiments that can be used by policy makers and land managers to make informed decisions about policies and production practices that apply to erodible landscapes.
Milestones
(2009): Manual of methods and procedures for phase six ; Website revisions and enhancements to promote committee coordination and communication between annual meetings.(2010): Site selection and site characterization
(2011): Develop proposal for external competitive funding using preliminary project data
(2012): Completion of sample and laboratory analyses
(2013): Workshops and meetings
(2014): Project Completion
Projected Participation
View Appendix E: ParticipationOutreach Plan
Results will be presented in refereed publications and in posters and symposia at National Meetings of the American Society of Agronomy, Soil Science Society of America, and the Soil and Water Conservation Society. We will also provide the information to collaborating agencies such as the NRCS and various federal, state, and local agencies. Additionally, committee members will extend this information at workshops, local field days, field tours, and through preparation of fact sheets.
Organization/Governance
The Regional Technical Committee will follow the operational procedures listed in the State Agricultural Experiment Directors, CSREES and ESCOP document entitled "Guidelines for Multistate Research Activites" revised and dated April 2002. The voting membership of the Regional Technical Committee includes one representative from each cooperating agricultural experiment station or institution appointed by the director and a representative of each cooperating USDA-ARS research unit or location. The administrative advisor and the CSRS representative are non-voting members. All voting members of the Technical Committee are eligible for office. The offices of the Regional Technical Committee include the Chair, the Vice-Chair and the Secretary. These officers will be elected annually at the Technical Committee meeting, and they may succeed themselves. The duties of the Technical Committee are to coordinate work activities related to the project. The Chair, in accord with the Administrative Advisor, will notify the Technical Committee of the time and place of the meeting will prepare the agenda and preside at meetings of the Technical Committee and Executive Committee. He or she is responsible for preparing the annual progress report and coordinating the preparation of regional reports. The Vice-Chair assists the Chair in all functions and the Secretary records the minutes and performs other duties assigned by the Technical Committee or Administrative Advisor. The Chair appoints subcommittees as needed. Annual meetings will be held by the Technical Committee, as authorized by the Administrative Advisor, for the purpose of conducting business related to the project.
Literature Cited
Allmaras, R.R., D.R. Linden and C.E. Clapp. 2004. Corn-residue transformations into root and soil carbon as related to nitrogen, tillage, and stover management. Soil Sci. Soc. Am. J. 68: 1366-1375. ASAE, 2000. ASAE Standard: ASAE S313.3 Soil cone penetrometer. p. 832-833. In. Agricultural Engineers Yearbook of Standards. ASAE. St. Joseph, MI. Bajracharya, R.M. and R. Lal. 1998. Crusting effects on erosion processes under simulated rainfall on a tropical Alfisol. Hydrol. Processes 12:1927-1938. Bajracharya, R., R. Lal and J.M. Kimble. 2000a. Diurnal and seasonal CO2-C flux from soil as related to erosion phases in central Ohio. Soil Sci. Soc. Am. J. 64:286-293. Bajracharya, R., R. Lal and J.M. Kimble. 2000b. Erosion phase effects on CO2 concentration and CO2 flux from an Alfisol. Soil Sci. Soc. Am. J. 64:694-700. Ball, B.C., and P. Schjonning. 2002. Air permeability. p. 1141-1158. In J.H. Dane and G.C. Topp (ed.) Methods of soil analysis Part 4: Physical methods. Soil Sci. Soc. of Am. Book Series: 5. Madison, WI. Barber, S.A. 1979. Corn residue management and soil organic matter. Agron. J. 71:625-627. Baumhardt, R.L., P.W. Unger and T.H. Dao. 2004. Seedbed surface geometry effects on soil crusting and seedling emergence. Agron. J. 96:1112-1117. Beale, O.W., G.B. Nutt, and T.C. Peele. 1955. The effects of mulch tillage on runoff, erosion, soil properties, and crop yields. Soil Sci. Soc. Am. Proc. 19:244-247. Black, A.L. 1973a. Crop residue, soil water, and sol fertility related to spring wheat production and quality after fallow. Soil Sci. Soc. Am. Proc. 37:754-758. Black, A.L. 1973b. Soil property changes associated with crop residue management in a wheat-fallow rotation. Soil Sci. Soc. Am. Proc. 37:943-946. Blanco-Canqui, H. and R. Lal. 2007a. Impact of long-term wheat straw management on soil hydraulic properties under no-tillage. Soil Sci. Soc. Am. J. 71:1166-1173. Blanco-Canqui, H. and R. Lal. 2007b. Soil structure and organic carbon relationships following 10 years of whet straw management in no-till. Soil & Till. Res. 95:240-254. Blanco-Canqui, H., R. Lal, F. Sartori and R.O. Miller. 2007. Changes in soil aggregate properties and organic carbon following conversion of agricultural lands to fiber farming. Soil Sci. 172:553-564. Blanco-Canqui, H., R. Lal, W.M. Post, R.C. Izaurralde and L.B. Owens. 2006. Corn Stover Impacts on Near-Surface Soil Properties of No-Till Corn in Ohio. Soil Sci. Soc. Am. J. 70:266-278. Blanco-Canqui, H., R. Lal, W.M. Post, R.C. Izaurralde, M.J. Shipitalo. 2007. Soil hydraulic properties influenced by corn stover removal from no-till corn in Ohio. Soil & Tillage Res. 92:144-155. Bohlen, P.J., R.W. Parmelee, D.A. McCarney and C.A. Edwards. 1997. Earthworm effects on carbon and nitrogen dynamics of surface litter in corn agroecosystems. Ecol. Applic. 7:1341-1349. Bouwman, A.F. (ed). 1990. Soils and the Greenhouse Effect. J. Wiley and Sons, Chichester, U.K. Busscher, W.J. and P.J. Bauer. 2003. Soil strength, cotton growth and lint yield in a southeastern USA coastal loamy sand. Soil Till. Res. 74:151-159. Butt, K.R., M.J. Shipitalo, P.J. Bohlen, W.M. Edwards and R.W. Parmelee. 1999. Long-term trends in earthworm populations of cropped experimental watersheds in Ohio, USA. Pedobiologia 43:713-719. Clothier, B., and D. Scotter. 2002. Unsaturated water transmission parameters obtained from infiltration. p. 879-898. In J.H. Dane and G.C. Topp (ed.) Methods of soil analysis Part 4: Physical methods. Soil Sci. Soc. of Am. Book Series: 5. Madison, WI. Crosson, P.R. 1984. New perspectives on soil conservation policy. J. Soil Water Cons. 30:222-225. Crosson, P.R. 1986. Agriculture and the Environment (T. Phipps, P. Crosson, and K. Price, eds.), Resources for the Future, Washington, DC. pp. 35-73. Dabney, S.M., G.V. Wilson, K.C. McGregor and G.R. Foster. 2004. History, residue, and tillage effects on erosion of loessial soil. Trans. ASAE 47:767-775. den Biggelaar, C., R. Lal, K. Wiebe, and V. Breneman. 2001. Soil erosion impacts on crop yields in North America. Advances in Agronomy 72(1). pp. 1-52. Doran, J.W., and A. J. Jones.1996. Methods for assessing soil quality. Soil Science Society of America. SSSA Special Publ. No. 49: 410 p. Duiker, S.J. and R. Lal. 1999. Crop residue and tillage effects on C sequestration in a Luvisol in central Ohio. Soil Tillage Res. 52:73-81. Ela, S.D., S.C. Gupta and W.J. Rawls. 1992. Macropore and surface seal interactions affectin water infiltration into soil. Soil Sci. Soc. Am. J. 56:714-721. Findeling, A., S. Ruy and E. Scopel. 2003. Modeling the effects of a partial residue mulch on runoff using a physically based approach. J. Hydrol. 275:49-66. Food and Agriculture Organization (FAO). 2001. Soil carbon sequestration for improving land management. World Resource Rep. #96. p. 62. Published by Food and Agriculture Organization for the United Nations. Frye, W.W., O.L. Bennett, and G.J. Buntley. 1985. Restoration of crop productivity on eroded or degraded soils. p. 335-355. In R.F. Follett and B. A. Stewart (eds). Soil Erosion and Crop for Productivity. ASA, 677 South Segoe Road, Madison, WI 53711, USA. Frye, W.W., S.A. Ebelhar, L.W. Murdock, and R.L. Blevens. 1982. Soil erosion effects on properties and productivity of two Kentucky soils. Soil Sci. Soc. Am. J. 46:1051-1055. Gregorich, E.G., K.J. Greer, D.W. Anderson, and B.C. Liang. 1998. Carbon distribution and losses: erosion and deposition effects. Soil Tillage Res. 47:291-302. Grover, B.L. 1955. Simplified air permeameters for soil in place. Soil Sci. Soc. Am. Proc. 19:414-418. Grunwald, S., B. Lowery, D.J. Rooney, and K. McSweeeney. 2001. Profile cone penetrometer data used to distinguish between soil materials. Soil Tillage Res. 62:27-40. Gupta, S.C., E.C. Schneider, W.E. Larson and A. Hadas. 1987. Influence of corn residue on compression and compaction behavior of soils. Soil Sci. Soc. Am. J. 51:207-212. Hall, G.F. 1983. Pedology and geomorphology. p. 117-140. In L.P. Wilding, N.E. Smeck, and G.F. Hall (eds). Pedogenesis and Soil Taxonomy. Elsevier, New York. Harris, R. F., D. L. Karlen and D. J. Mulla. 1996. A conceptual framework for assessment and management of soil quality and health, Chapter 4, p. 61-82. In Doran, J. W., and A. J. Jones. (eds.). Methods for Assessing Soil Quality. (1st ed.) Soil Sci. Soc. Am. Pub. No 49. Soil Sci. Soc. Am., Madison, WI. Hooker, B.A., T.F. Morris, R. Peters and Z.G. Cardon. 2005. Long-term effects of tillage and corn stalk return on soil carbon dynamics. Soil Sci. Soc. Am. J. 69:188-196. Johnson, J.M.F., D. Reicosky, B. Sharratt, M. Lindstrom, W. Voorhees and L. Carpenter-Boggs. 2004. Characterization of soil amended and the by-product of corn stover fermentation. Soil Sci. Soc. Am. J. 68:139-147. Karlen, D.L., N.C. Wollenhaupt, D.C. Erbach, E.C. Berry, J.B. Swan, N.S. Eash and J.L. Jordahl. 1994. Crop residue effects on soil quality following 10 years of no-till corn. Soil Till. res. 31:149-167. Khan,S.A., R.L. Mulvaney,T.R.Ellsworth and C.W.Boast 2007.The myth of nitrogen fertilization for soil carbon sequestraion .J.Env.Qual.36:1821-1832. Kim, S. and B.E. Dale. 2004. Gloal potential bioethanol production from wasted crops and crop residues. Biomass Bioenergy 26:361-375. Kladivko, E.J. 1994. Residue effects on soil physical properties. p. 123-162. In P.W. Unger (ed.) Managing agricultural residues. Lewis Publishers, Boca Raton, FL. Kreznor, W. R., K. R. Olson, W. L. Banwart, and D. L. Johnson. 1989. Soil, landscape, and erosion relationships in a Northwest Illinois. Soil Sci. Soc. Am. J. 53: 1763-1771. Kreznor, W. R., K. R. Olson, and D. L. Johnson. 1992. Field evaluations of methods to estimate soil erosion. Soil Sci. 153:69-81. Lal, R. 1995. Global soil erosion by water and carbon dynamics. p. 131-142. In R. Lal, J.M. Kimble, E. Levine and B.A. Stewart (eds). Soils and Global Change. CRC/Lewis Publishers, Boca Raton, FL. Lal, R. 1999. (ed). Soil Quality and Soil Erosion. CRC Press. Boca Raton, FL. 329p. Lal, R. 2003. Soil erosion and the global carbon budget. Env. Intl. 29:437-450. Lal, R., D. De Vleeschauwer and R.M. Nganje. 1980. Changes in properties of a newly cleared tropical Alfisol as affected by mulching. Soil Sci. Soc. Am. J. 44:827-833. Lal, R., J.M. Kimble, E. Levine and B.A. Stewart (eds). 1995a. Soils and Global Change. CRC/Lewis Publishers, Boca Raton, FL. 440p. Lal, R., J.M. Kimble, E. Levine and B.A. Stewart (eds). 1995b. Soil Management and Greenhouse Effect. CRC/Lewis Publishers, Boca Raton, FL. 385p. Langdale, G.W., H.P. Denton, A.W. White, Jr., J.W. Gilliam, and W.W. Frye. 1985. Effects of soil erosion on crop productivity of Southern soils. p. 251-269. In R. J. Follett and B.A. Stewart (eds.) Soil Erosion and Crop Productivity. Am. Soc. Agron., 677 S. Segoe Road, Madison, WI. Larson, W.E., C.E. Clapp, W.H. Pierre, and Y.B. Morachan. 1972. Effects of increasing amounts of organic residues on continuous corn. II. Organic carbon, nitrogen, phosphorus and sulfur. Agron. J. 64:204-208. Larson, W.E., R.F. Holt and C.W. Carlson. 1978. Residues for soil conservation. p. 1-15. In W.R. Oschwald (ed.) Crop residue management systems. Spec. Pub. 21. ASA, Madison, WI. Lindstrom, M.J. E.L. Skidmore, S.C. Gupta and C.A. Onstad. 1979. Soil conservation limitations on removal of crop residues for energy production. J. Environ. Qual. 8:533-537. Lindstrom, M.J., T.E. Schumacher, A.J. Jones, and C. Gantzer. 1992. Productivity index model comparison for selected north central region soils. J. Soil Water Conserv. 47:491-494. Lindstrom, M.J., J.A. Schumacher, and T.E. Schumacher. 2000. TEP: A tillage erosion prediction model to calculate soil translocation rates from tillage. J. Soil Water Conserv. 55:105-108. Loll, P., P. Moldrup, P. Schjønning and H. Riley. 1999. Predicting saturated hydraulic conductivity from air permeability: application in stochastic water infiltration modeling. Water Resour. Res. 35:2387-2400. Lowery, B., T.M. Lillesand, D.H. Mueller, P. Weiler, F.L. Scarpace, and T.C. Daniel. 1984. Quantitative determination of crop residue using a microdensitometer. J. Soil Water Conserv. 39: 402-403. Lowery, B., and J.E. Morrison, Jr. 2002. Soil penetrometers and penetrability. p. 363-388. In J.H. Dane and G.C. Topp (ed.) Methods of soil analysis Part 4: Physical methods. Soil Sci. Soc. of Am. Book Series: 5. Madison, WI. Maiorana, M., A. castrignano and F. Fornaro. 2001. Crop residue management effects on soil mechanical impedance. J. Agric. Eng. Res. 79:231-237. Malo, D.D., B.K. Worcester, D.K. Cassel, and K.D. Matzdorf. 1974. Soil-landscape relationships in a closed drainage system. Soil Sci. Am Proc., 38: 813-818. Mankin, K.R., A.D. Ward and K.M. Boone. 1996. Quantifying changes in soil physical properties from soil and crop management: a survey of experts. Trans. ASAE 39:2065-2074. McVay, K.A., J.A. Budde, K. Fabrizzi, M.M. Mikha, C.W. Rice, A.J. Schlegel, D.E. Peterson, D.W. Sweeney and C. Thompson. 2006. Management effects on soil physical properties in long-term tillage studies in Kansas. Soil Sci. Soc. Am. J. 70(2):434-438. Minitab. 2000. Users Guide 2: Data Analysis and Quality Tools. Minitab Inc. Mochizuki, M.J., A. Rangarajan, R.R. Bellinder, H.M. van Es and T. Bjorkman. 2008. Rye mulch management affects short-term indicators of soil quality in the transition to conservation tillage for cabbage. Hortsci. 43(3):862-867. Morachan, Y.B. W.C. Moldenhauer and W.E. Larson. 1972. Effects of increasing amounts of organic residues on continuous corn: I. Yields and soil physical properties. Agron. J. 64:199-203. National Resource Inventory. 1997. 2000 update. USDA, NRCS Nelson, D.W. and L.E. Sommers. 1982. Total carbon, organic carbon, and organic matter. Chapter 29. p. 539-579. In A.L. Page (ed). Methods of Soil Analysis Part 2, Chemical and Microbiological Properties (2nd edition). Agronomy Monograph No. 9. American Society of Agronomy. Madison, WI. Nelson, R.G. 2002. Resource assessment and removal analysis for corn stover and wheat straw in the Eastern and Midwestern United States-Rainfall and wind-induced soil erosion methodology. Biomass Bioenergy 22:349-363. Olson, K.R. 1992. Soil physical properties as a measure of cropland productivity. In Proc. of the Soil Quality Standards Symposium. USDA. Forest Service. WO-WSA-2. Olson, K. R., S. R. Phillips, and B. K. Kitur. 1994. Identification of eroded phases of an Alfisol. Soil Sci. 157:108-115. Olson, K. R., R. Lal, and L.D. Norton. 1994. Evaluation of methods to study soil erosion-productivity relationships. J. Soil Water Conserv. 49:586-590. Or, D. and T.A. Ghezzehei. 2002. Modeling post-tillage soil structural dynamics: A review. Soil Till. Res. 64:41-59. Ovalles F.A. and M.E. Collins. 1986. Soil-landscape relationships and soil variability in north central Florida. Soil Sci. Soc. Am. J. 50: 401-408. Parkin, T.B. and T.C. Kaspar, 2003. Temperature controls on diurnal carbon dioxide flux: Implications for estimating soil carbon loss. Soil Sci. Soc. Am. J. 67:1763-1772. Phillips, J.A. and E.J. Kamprath. 1973. Soil fertility problems associated with land farming on the Coastal Plain. J. Soil Water Conserv. 18:69-73. Pikul Jr., J.L. and R.R. Allmaras. 1986. Physical and chemical-properties of a Haploxeroll after 50 years of residue management. Soil Sci. Soc. Am. J. 50:214-219. Pikul, J.L., R.C. Schwartz, J.G. Benjamin, R.L. Baumhardt and S. Merrill. 2006. Cropping system influences on soil physical properties in the Great Plains. Renewable Agric. and Food Sys. 21(1):15-25. Pimentel, D., C. Harvey, P. Resosudarmo, K. Sinclair, D. Kurz, M. McNair, S. Crist, L. Shpritz, L. Fitton, R. Saffouri, and R. Blair. 1995. Environmental and economic costs of soil erosion and conservation benefits. Science 267:1117-1123. Pimentel, D. 1992. Energy inputs in production agriculture. Energy World Agric. 6:13-29. Pimentel, D., E.C., Terhune, R. Dyson-Hydson, S. Rochereau, R. Smais, E.A. Smith, D. Deuman, D. Reifschneider, and M. Shepard. 1976. Land degradation: effects on food and energy resources. Science 194:149-155. Reuss, J. O. and R.E. Campbell. 1961. Restoring productivity to leveled land. Soil Sci. Soc. Am. Proc. 25:302-304. Riecken, F.F. and E. Poetsch. 1960. Genesis and classification considerations of some prairie-formed soil profiles from local alluvium in Adair County, Iowa. Proc. Iowa Acad. Sci. 67: 268-276. Rolston, D.E. 1986. Gas diffusivity. p. 1089-1102. In. A. Klute (ed.) Methods of Soil Analyses, Part 1. Physical and Mineralogical Methods. Agronomy Monograph 9, ASA, Madison, WI. Rooney, D. and B. Lowery. 2000. A profile cone penetrometer for mapping soil horizons. Soil Sci. Soc. Am. J. 64:2136-2139. Ruhe, R.V. Geomorphology. Houghton Mifflin, Boston. 246 pp. Salinas-Garcia, J.R., A.D. Báez-González, M. Tiscareno-López and E. Rosales-Robles. 2001. Residue removal and tillage interaction effects on soil properties under rain-fed corn production in Central Mexico. Soil Till. Res. 59:67-79. Schonbeck, M.W. and G.K. Evanylo. 1998. Effects of mulches on soil properties and tomato production II. Plant-available nitrogen, organic matter input, and tilth-related properties. J. Sustain. Agric. 13:83-100. Schumacher, T.E., M.J. Lindstrom, D.L. Mokma and W.W. Nelson. 1994. Corn yield-erosion relationships of representative loess and till soils in the North-Central United States. Soil Water Conserv. 49:343-348. Schumacher, T.E., M.J. Lindstrom, J.A. Schumacher, and G.D. Lemme. 1999. Modeling spatial variation in productivity due to tillage and water erosion. Soil Tillage Res. 51: 331-339. Schumacher, T.E., M.J. Lindstrom, J.A. Schumacher, D.C. Reicosky and D.D. Malo. 2003. Carbon distribution in a hummocky landscape: an estimate of surface translocation by tillage and water. Soil Water Conservation Abstracts. p. 75. Sharpley, A.N. 1985. The selective erosion of plant nutrients in runoff. Soil Sci. Soc. Am. J. 49:1527-1534. Sharratt, B.S. 1996. Tillage and straw management for modifying physical properties of a subarctic soil. Soil Till. Res. 38:239-250. Sharratt, B.S. 2002. Corn stubble height and residue placement in the northern US Corn Belt Part I. Soil physical environment during winter. Soil Till. Res. 64:243-252. Shaver, T.M., G.A. Peterson, L.R. Ahuja, D.G. Westfall, L.A. Sherrod and G. Dunn. 2002. Surface soil physical properties after twelve years of dryland no-till management. Soil Sci. Soc. Am. J. 66:1296-1303. Shipitalo, M.J. and K.R. Butt. 1999. Occupancy and geometrical properties of Lumbricus terrestris L-burrows affecting infiltrations. Pedobiologia 43:782-794. Simpson, T.W., A.N. Sharpley, R.W. Howarth, H.W. Paerl, and K.R. Mankin. 2008. The new gold rush: Fueling ethanol production while protecting water quality. J. Environ. Qual. 37:318-324. Singh, B., D.S. Chanasyk and W.B. McGill. 1996. Soil hydraulic properties of an Orthic Black Chernozem under long-term tillage and residue management. Can. J. Soil Sci. 76:63-71. Skidmore, E.L., J.B. Layton, D.V. Armbrust and M.L. Hooker. 1986. Soil physical-properties as influenced by cropping and residue management. Soil Sci. Soc. Am. J. 50:415-419. Somerville,C. 2006. The billion-ton biofuel vision.Science 312:1277. Strudley, M.W., T.R. Green and J.C. Ascough. 2008. Tillage effects on soil hydraulic properties in space and time: State of the science. Soil & Tillage Res. 99(1):4-48. U.S. Congress.1985. Food Security Act of 1985. P.L. 99-198. 99th Congress, Washington, D.C. U.S. Department of Agricultlure-Natural Resources Conservation Service. 1996. Soil quality indicators: Soil crusts. http://soils.usda.gov/sqi/files/sq_sev_1.pdf (verified 29 Sept. 2005). Walker P.H. and R.V. Ruhe.1968. Hillslope models and soil formation. II. Closed systems. Trans. 9th Int. Congress Soil Sci., Adelaide, p. 561-568. Wells, R.R., D.A. DiCarlo, T.S. Steenhuis, J.-Y. Parlange, M.J.M. Römkens and S.N. Prasad. 2003. Infiltration and surface geometry reatures of a swelling and following successive simulated rainstorms. Soil Sci. Soc. Am. J. 67:1344-1351. Wilhelm, W.W., J.W. Doran and J.F. Power. 1986. Corn and soybean yield response to crop residue management under no-tillage production systems. Agron. J. 78:184-189. Wilhelm, W.W., J.M.F. Johnson, J.L. Hatfield, W.B. Voorhees and D.R. Linden. 2004. Crop and soil productivity response to corn residue removal: A literature review. Agron. J. 96:1-17. Wilts, A.R., D.C. Reicosky, R.R. Allmaras and C.E. Clapp. 2004. Long-term corn residue effects: harvest alternatives, soil carbon turnover, and root-derived carbon. Soil Sci. Soc. Am. J. 68:1342-1351. Wooding, R.A. 1968. Steady infiltration from a shallow circular pond. Water Resour. Res. 4:1259-1273.