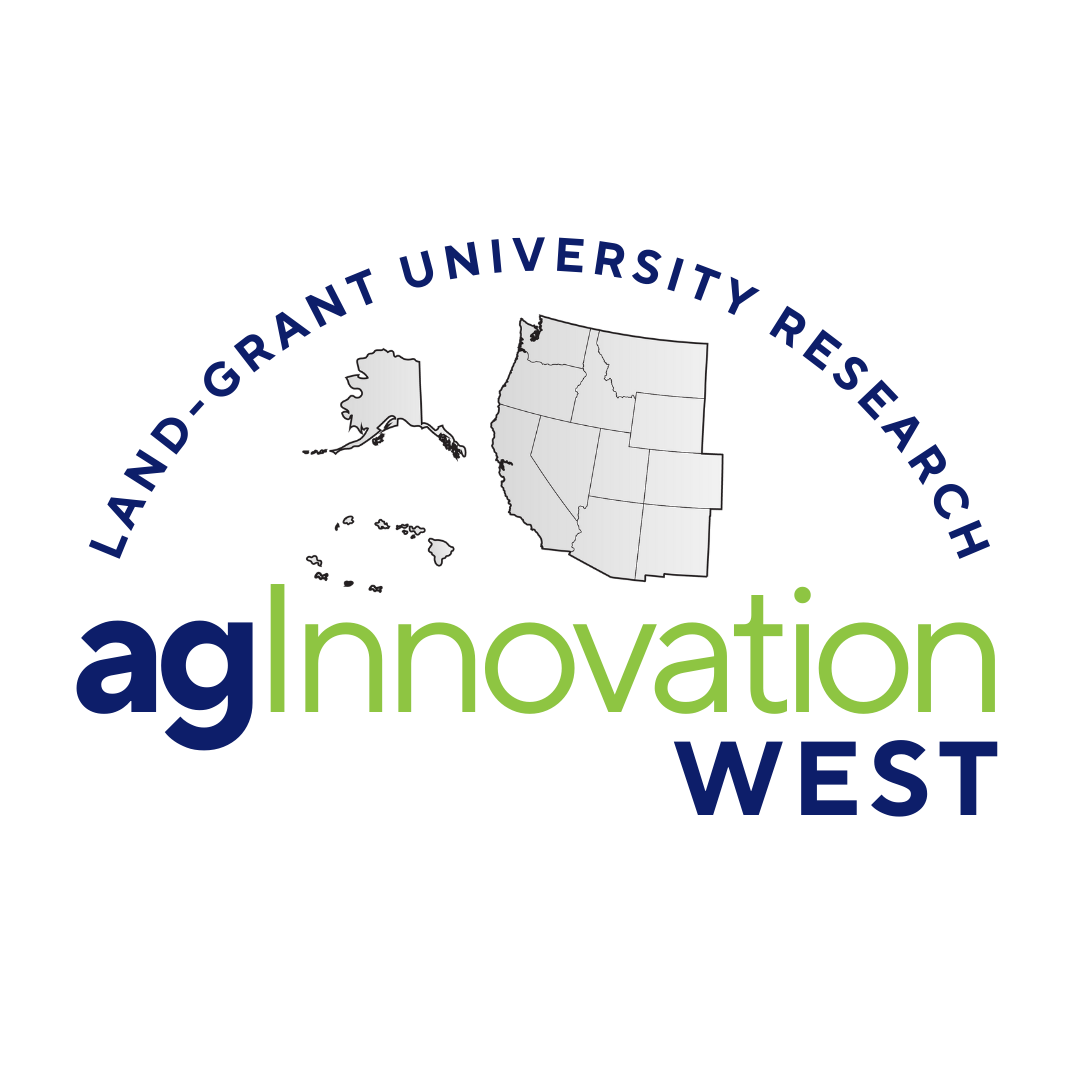
W5147: Managing Plant Microbe Interactions in Soil to Promote Sustainable Agriculture
(Multistate Research Project)
Status: Active
W5147: Managing Plant Microbe Interactions in Soil to Promote Sustainable Agriculture
Duration: 10/01/2023 to 09/30/2028
Administrative Advisor(s):
NIFA Reps:
Non-Technical Summary
Statement of Issues and Justification
A. The Problem
Soilborne diseases continue to be a serious problem in US agriculture. They are caused primarily by fungi and nematodes, and attack the root systems, reducing the ability of plants to take up water and nutrients. They are difficult to diagnose, because above-ground symptoms may not be distinctive, and could be mistaken for nutrient deficiencies or drought. Unlike foliar diseases, they are difficult to manage with chemicals. They form survival structures in the soil that are resistant to chemicals, and it is near impossible to treat all the soil, except with fumigation, which can result in environmental degradation. Unlike with biotrophic diseases like rusts, there is little genetic resistance to soilborne diseases in available and industry accepted crop cultivars. Although some exceptions exist (i.e., Fusarium wilt, Fusarium oxysporum), in most cases host resistance to soilborne diseases is multigenic and difficult to breed for.
Economic Costs Due to Soilborne Plant Pathogens
According to the Food and Agriculture Organization (FAO) of the United Nations, plant diseases and pests reduce agricultural production by 20-40%, which represents a global cost of approximately 220 billion USD for diseases alone (Lazo, 2023). As such, 2020 was declared the International Year of Plant Health. For root diseases of mature crops, there are few effective and economical post-planting strategies for control. About 90% of the 2000 major diseases of the principal crops in the U.S. are caused by soilborne plant pathogens, most of which are fungi or fungus-like organisms (Panth et al., 2020). Many pathogens have a soil- or debris-borne over seasoning stage. In many cases, resistance to soilborne diseases is quantitative and specific pathogen race structures and population diversity parameters remain understudied.
Table 1. Crop production (million metric tons) for the top 10 crops (excluding vegetables) in the U.S. in 2021 (NASS, 2023).
Crop |
Production |
Rank |
Crop |
Production |
Rank |
Corn |
383.9 |
1 |
Sorghum |
13.7 |
6 |
Soybeans |
120.7 |
2 |
Rice |
9.7 |
7 |
Wheat |
44.8 |
3 |
Cotton |
3.8 |
8 |
Sugarbeets |
33.3 |
4 |
Peanuts |
2.9 |
9 |
Sugarcane |
29.8 |
5 |
Barley |
2.6 |
10 |
As examples, certain commodities quantify and report yield loss data to all categories of diseases including those caused by soilborne pathogens. Corn data from 2016 to 2019 showed an average yearly loss of 9.6 million metric tons (Mmt) (2.4% of the 2021 production value; Table 1) to soilborne diseases including Fusarium stalk rot (F. verticilliodes, others) (5.1 Mmt yr-1), nematodes (1.7 Mmt yr-1), Gibberella stalk rot (Gibberella zeae) (1.3 Mmt yr-1), seedling blights (0.9 Mmt yr-1), and root rots (0.7 Mmt yr-1) (Mueller et al., 2020). Likewise, soybean data from 2015 to 2019 showed an average yearly loss of 3.9 Mmt (3.2% of the 2021 production value; Table 1) to soilborne diseases including seedling diseases (1.29 Mmt yr-1), white mold (Sclerotinia sclerotiorum) (1.10 Mmt yr-1), sudden death syndrome (Fusarium virguliforme) (1.01 Mmt yr-1), Phytophthora root rot (Phytophthora sojae, others) (0.66 Mmt yr-1), and charcoal rot (Macrophomina phaseolina) (0.04 Mmt yr-1) throughout the northern and southern soybean- producing states. Detailed studies on the wheat crops in the Pacific Northwest had documented loss due to Pythium, Fusarium, and Rhizoctonia. For 2021, the cotton disease committee (Cotton, Inc.) estimated losses in 480 lb bales to Fusarium wilt, Verticillium wilt (Verticillium dahliae), cotton root rot (Phymatotrichopsis omnivora), and seedling diseases (several fungi) to be 64800 bales (0.35%), 67600 bales (0.37%), 26500 bales (0.14%), and 146100 bales (0.80%), respectively.
New or re-emerging soilborne diseases are a constant threat to crop production in the United States. Such issues arise through pathogen introductions, changes in host germplasm, and altered control measures. For example, wheat blast is a new disease in South America (Brazil) caused by a strain of the pathogen Magnaporthe oryzae. It has recently been detected in Bangladesh, but is not yet present in the United States. Macrophomina crown rot (Macrophomina phaseolina) and Fusarium wilt in strawberries have become new problems, because of the loss of methyl bromide. In corn, tar spot (Phyllachora maydis) has emerged and caused disease losses in the U.S. since 2018 (Kleczewski et al., 2021). In soybean, Xylaria necrophora has emerged as a root rot and taproot decline pathogen in the southern U.S. (Garcia-Aroca et al., 2021) since ~2017. Several of the top 15 restricted, invasive quarantine pathogens listed by APHIS are soilborne and could represent biosecurity risks.
New invasive species have been discovered in North America in the last fifteen years, including Phytophthora ramorum, cause of sudden oak death, and Phytophthora tenticulata, have decimated native ecosystems in California. In natural ecosystems, once they become established, these pathogens cannot be easily managed. Laurel wilt, caused by Raffaelea lauricola and vectored by exotic ambrosia beetles, threatens the native laurels of the East Coast and the avocado industry in Florida and California. Boxwood blight, caused by Cylindrocladium buxicola, discovered in the US in 2011, has become endemic in 10 states.
Finally, the changing climate represents a challenge and an opportunity to address soilborne pathogen problems. Changing climate will result in more plant stress, drought conditions, salinity or in some cases a wetter climate, which will predispose plants to more disease. Models based on global field surveys have shown that increasing soil temperature may increase the abundance of soilborne fungal pathogens (Delgado-Baquerizo et al. 2020). Further, Chaloner et al. (2021) project that as climate changes, higher latitudes will see greater yields, but pathogen turnover is projected to increase in some of the most productive swaths of global agricultural land. This includes the central Great Plains of the United States. Charcoal rot, a drought and high-temperature disease, was in the top 10 soybean diseases for the southern United States every year from 2015-2019, but had appeared in the top 10 diseases of the northern U.S. more often (3 of 5 yrs) during this period compared to previous disease loss reports (≤ 2 of 5 yrs).
Cost to the environment
The cost of soilborne plant pathogens to society and the environment far exceeds the direct costs to growers and consumers. The use of chemical pesticides to control soilborne pathogens has caused significant changes in air and water quality, altered natural ecosystems resulting in direct and indirect effects on wildlife, and caused human health problems. For example, methyl bromide, a fumigant used to control soilborne diseases, has become notorious in recent years for contributing to the depletion of the ozone layer. The planned ban on production and importation of this product has been repeatedly delayed by a lack of cost-effective alternatives, and there remains an intensive search for replacement control methods. This fumigant was to be totally banned by 2005, but there are still a few critical use exemptions for the U.S. Larger buffers and restriction zones are needed for many pesticides. Soil fumigants are major contributors to volatile organic compounds affecting air quality, especially in the Central and Imperial Valley of California. Development of fungicide resistance continues to be a problem with the newer generation of reduced-risk fungicides with specific modes of action, such as the strobilurins. Additionally, plants evolved in the presence of microorganisms and are dependent on them in order to carry out many activities necessary for growth and reproduction. Thus, long-term chemical applications may permanently alter the microbial community structure sufficiently such that sustainable agriculture may be impossible.
B. The Solution.
The future of sustainable agriculture in the U.S. will increasingly rely on the integration of biotechnology with traditional agricultural practices. Although genetic engineering promises enhanced yields and disease resistance, it is also important to recognize that plants exist in intimate associations with microorganisms, some of which cause plant disease while others protect against disease. Identifying, understanding and utilizing microorganisms or microbial products to control plant disease and enhance crop production are becoming more central parts of sustainable agriculture. Biological control or biologically-based pest management (BBPM) has the potential to control crop diseases while causing no or minimal detrimental environmental impact. For this proposal, we define biological control as the manipulation of microbial populations through cultural, physical or biological means including plant mechanisms. Some of the benefits of utilizing microorganisms include:
- Reduced dependence on chemical pesticides, which is important because of expanding demand for organic produce and increasing costs of such petroleum-based inputs
- Lack of development of pathogen resistance to biological control organisms
- Lower regulatory costs of registration
- Faster reentry times after application
- More selective action against pathogens and not against beneficial organisms
- Biodegradability of microbial pesticides and their by-products
- Reduced danger to humans or animals
- Improvement of soil quality and health
- Increased food safety
- Management of diseases in natural ecosystems
- Improve plant productivity via controlling abiotic stress
- Adaptation to climate change, as pathogen distributions shift
- Increased N use efficiency and reduced N and P contamination of waterways and oceans
In the last five years, there has also been an increased awareness of soil health and the importance of microbes in providing benefits to plant health and productivity. Over the last 10 years, almost 30,000 papers have been published on the topic of soil health. There is an established non-profit (Soil Health Institute, https://soilhealthinstitute.org). States such as Washington have funded a soil health institute via legislative appropriations (Washington State Department of Agriculture, 2023). While NRCS has developed on-line resources to inform growers about soil health, there are no validated protocols for assessing soil health for all cropping systems and growers are clamoring for tests to help them determine their soil’s health. Additionally, THERE IS A LACK OF UNDERSTANDING ABOUT HOW SPECIFIC MICROBIAL GROUPS DRIVE SOIL HEALTH. Research proposed BY THIS PROJECT addresses these knowledge gaps and is ADVANCING THE FIELD.
Objectives
To address this problem, this project will propose four objectives
- Objective 1. Biocontrol via one or a small number of microbes and/or their products
- Objective 2. Biocontrol via microbial communities and soil health
- Objective 3. Creating and testing disease management and/or soil health strategies
- Objective 4. Extension and outreach
How this project fits the goals of USDA and REE
This project fits REE Action Goal Framework Goal 1: Sustainable Intensification of Agricultural Production. In 2017, USDA also issued a set of goals, and this overlaps with USDA GOALS 2 & 3. GOAL 2: Maximize the Ability of American Agricultural Producers To Prosper by Feeding and Clothing the World and GOAL 3. Promote American Agricultural Products and Exports. This entire project is designed to help farmers and growers manage soilborne pathogens in an environmentally sustainable way, thus directly impacting the large agricultural industry of the United States.
C. Addressing the Needs of the Stakeholders
- The Biopesticide Industry.
Biopesticides are the fastest-growing crop protection market sector exhibiting substantial growth over the past three decades as the market has grown into a multibillion-dollar business. Demand for biopesticides has continued to expand dramatically in the last five to ten years. In the 5 years since our previous proposal, the global market for biopesticides has doubled from $1.5 billion to $3 billion US (Biological Pesticide Products Industry Alliance, 2023) and is expected to triple by 2027 (Batista and Singh, 2021; Sessitsch et al. 2018). Recent policy changes, such as the new Green Deal of the European Union, have expanded the biological product market. The Biological Pesticide Products Industry Alliance, established in 2001, had 31 member companies in 2006, 65 members in 2012 and now has over 120 members. The International Biocontrol Manufacturers Association had 130 companies marketing microbial biocontrol agents in 2012. But now there are approximately 2530 biopesticide manufacturers (not including China and India) with about 98 of those in the Americas and 91 in Europe. This segment of the industry is expected to grow between 15% and 20% annually (http://www.ibma-global.org). This growth has been driven by expanding organic markets as well as increased public sensitivity to the risks and hazards of chemical pesticides. From 2008 to 2012, 15 microbial active ingredients have been registered by EPA. From 2012 to 2017, six Bacillus spp., two Trichoderma spp., one Streptomyces sp., one Pseudomonas sp. and one Muscodor sp. have entered the EPA regulatory process (EPA, 2017). In the last 5 years, there has been a concerted effort by larger companies, such as Syngenta, BASF, FMC, and Bayer, to develop inhouse expertise by partnering or acquiring smaller companies, to increase their investment in this field. For example, Bayer CropScience bought AgraQuest, acquiring Serenade and other Bacillus products.
Additionally, the recent development of next-generation sequencing technologies and capacity to analyze metagenomic data has led to a greater understanding of how soil microbial communities function and influence plant tolerance to biotic and abiotic stressors. This technology is presently being utilized by our members to gain insights into plant-microbe interactions and identify implications for biocontrol. In fact, there has been a significant increase in research publications on the use of soil inoculants and consortia of microbes for disease control (Canfora et al. 2021).
- The Microbiome Industry.
This is an industry that did not exist 15 years ago. The total agricultural microbial industry is estimated to increase by 17% yearly, with a value of $12 billion by 2026 (Research and Markets, 2018). Much of this growth is in “microbiome companies” such as Indigo, which has developed seed endophytes, AgBiome, Robigo, Inviao, and BioConsortia. Monsanto (now part of Bayer) invested a significant amount of money in microbiome research in the last 10 years. In addition, there are dozens of startup companies getting into the field. This involves a major paradigm shift.
Instead of just relying on developing biological control agents (BCAs) to apply to agriculture, the emphasis is to manage the rhizosphere and soil microbiome by management of the agricultural system, to promote disease suppression, nutrient uptake, and tolerance to abiotic stress. Nevertheless, many of these companies are using microbiome research to discover and market potential new inoculants. They are also marketing microbiome tests for farmers that would give them a diagnostic assessment of the soil health of fields, much in the same way companies have exploited this for the human gut microbiome. There is a large “probiotics” market for agriculture, but many of the claims made by developers of probiotics and soil health products and services are not based on sound research. Researchers in this project can provide sound, science-based evidence to help support adoption of products, services, and management practices that will be most valuable to growers.
- The Organic Industry
As is readily apparent from reading the popular press, consumers are demanding plentiful low cost but safe food while simultaneously requiring reduced use of chemical pesticides. This has been evident by the rapid growth of the organic food industry. In 2016, there were 5.1 million acres in organic production (NASS, 2023), almost double the acreage from 2008. In 2019, this number increased to 5,495,270 acres. with 16,585 farms. The total farm gate value of organic products in 2016 was $7.5 billion, compared to 3.5 billion in 2011 (NASS, 2023). By 2019, sales increased to 9.9 billion dollars. Organic food is now available from large retailers such as Walmart, Whole Foods, Kroger, and others.
Organically-grown crops require non-synthetic methods for management of diseases, and organic growers are seeking scientifically-based disease management methods. A 2015 survey by the Organic Farming Research Foundation (OFRF) identified disease management and soil health as one of the top five research priorities (Jenkins and Ory, 2016). In 2020, The Organic Farming Research Foundation (OFRF) and Organic Seed Alliance (OSA) released an update survey in 2020 for California growers.
Some of the production concerns that were identified include controlling disease pressure, minimizing adverse impacts of tillage on soil health, and adapting to climate change. One of the top research priorities is maintaining yield and soil health. Members of this project are doing this research. Many products that members of W-4147 have researched are certified as organic with the Organic Materials Review Institute (OMRI). During the last few years, more and more pesticides that control soilborne diseases have been taken off the market or regulated, including methyl bromide. Soilborne pathogens are well adapted to soil conditions, and once established are very difficult to eliminate. A classic example of a disease shift with the loss of methyl bromide has been the increased incidence of Fusarium wilt and charcoal rot of strawberries in California, which were not major problems 10 years ago. Even if chemical products are available, they are often too expensive to be economically practical and for many pathogens, chemical remedies have yet to be identified. Other approaches with great potential include the development of transgenic crops engineered with resistance genes to several pathogens. However, there is widespread public reluctance to accept these crops as evidenced by protests both here and in Europe. These concerns, combined with the natural ability of pathogens to overcome introduced resistance genes, has frustrated efforts to maximize this approach.
Why a Multi-State, Multi-Disciplinary Approach? This multistate project has a diverse group of researchers, with a long history of productivity and collaboration in the area of biological management of soilborne pathogens and diseases. Unlike some smaller groups that focus on one particular experiment or field trial across states, our group has fostered collaboration via the annual meetings. These often lead to new ideas and new collaborations, especially when we meet in person. This happens organically, rather than top down. This has resulted in large group projects (See Coordinated Agricultural Projects in Methods section). These are focused on a common crop (eg. potato, camelina, soybean) or pathogen (cyst nematode). This synergy would not be possible without the support of a multistate group. This group has been extremely productive over the last 5 years. We have published 362 peer reviewed publications, 23 book chapters, 3 theses and 66 conference proceedings/abstracts by our members, among many other activities. Few other multistate groups can show this level of productivity.
Goals and potential impact of the Project
The ultimate goals of this collaborative work of W-5147 are to:
- Provide society with a safe, low-cost food supply
- Reduce the environmental impact of soilborne disease control on ornamental, bioenergy, fiber and food crop production
- Protect natural and agroecosystems from invasive species
- Development of new industries and products for biologically based disease control
Related, Current and Previous Work
THE MICROBIOME
The newest area of biological control research is understanding and utilizing the communities of microorganisms found in plant- associated habitats such as soil, rhizosphere, and the endosphere. The microbial communities that inhabit these niches are called microbiomes. In this area of biological control research, instead of examining how one or a small number of microbes influences plant disease, large numbers of microorganisms, or an entire microbial community, are being examined to determine how they are affecting plant health and disease. Members of the W4147 have been leaders in this field. In the following, we describe several examples of this work.
Outreach to Stakeholders
One essential component of working in a new area of applied science is to inform the stakeholders about the field and keep them updated on the newest advances and capabilities. It is also important to get stakeholder input concerning how fundamental results might be translated into practical solutions, and to form collaborations with the stakeholders to test putative solutions in the field. Members of our group regularly interact with growers to foster these relationships, and they have also given numerous presentations on such topics. These presentations have covered fundamental topics such as what is a microbiome (Marks et al. 2020a). They have also covered topics such as how scientists are elucidating the relationships between microbiomes and plant health as well as between microbiomes and plant disease (Marks et al. 2020b, Evin et al. 2021).
Microbiome Methods
Another essential component of working in a new field is to create new methods. Since new fields are asking new questions, new methods are needed to answer them. Members of our group have been leaders in this area. The following are a few examples in chronological order.
Early on in the development of the field, one of our members published a new method that provided the simplest and fastest way to isolate DNA from soil. This method initiated a transformation in how DNA was isolated from environmental samples, contributing to the explosion of studies characterizing the vast and previously undescribed diversity of microbes inhabiting our planet. This soil DNA isolation method uses bead-beating-based cell lysis and column-based DNA purification (Borneman et al. 1996). Building on the commercial success of the kits that were based on these methods, a few of the researchers from BIO 101/MP Biomedicals started their own company, MoBio Laboratories, which became a leader in DNA and RNA isolation.
Another early advance was the development of a new method that provided a simple and rapid way to compare bacterial communities (Borneman and Triplett, 1997). Such analyses give researchers the ability to identify bacteria that are differentially abundant in two or more samples, which can be the key first step in identifying functionally important microbes (e.g. bacteria that cause or inhibit disease). Compared to the most commonly used method at the time (DGGE) (Muyzer et al. 1993), this new method (i) provided better taxonomic resolution, (ii) did not require specialized equipment, and (iii) it is still used today.
Another early advance was the development of a new method that provided a simple, fast, and culture-independent approach for identifying individual microbes that respond to specific chemical stimuli in environmental samples. This capability can be of considerable value in efforts to determine which microbes play key functional roles in complex ecosystems. By adapting a commonly used experimental strategy in mammalian biology, a culture-independent method was created that allowed the identification of microbes (bacteria, fungi, others) that actively divide in response to the addition of specific chemicals to an environmental sample (Borneman 1999). Here, a nucleotide analog (BrdU) and a specific chemical are added to an environmental sample such as soil and allowed to incubate. DNA is then isolated from that sample, the DNA that has incorporated the BrdU is captured using an antibody, and the resulting DNA is analyzed for its microbial community composition. An example experiment comparing uncaptured and antibody-captured DNA with a control soil and one amended with a solution of glucose and potassium phosphate, there were considerable differences between the treatment groups with the captured DNA but no differences with the uncaptured DNA.A more recent advance was the development of a new method for bacterial community analyses that provided increased taxonomic resolution. This feature is crucial for differentiating similar bacteria, and for determining if samples or subjects harbor functionally important bacteria (e.g. bacteria that cause or inhibit diseases such as IBD as described below). This was the first, Illumina-based, amplicon sequencing method that frequently enabled species- and sometimes strain-level resolution of bacteria. This increased resolution was obtained by analyzing the rRNA ITS region (Ruegger et al. 2014).
In very recent work, one of our members created new methods to analyze modern omics datasets. For example, they created an inexpensive and quantitative reduced-representation sequencing (qRRS) strategy, omeSeq, for describing microbial communities (Olukolu and Yencho, 2022). They have also developed new bioinformatic software that automates metagenomic analyses (Kuster et al. 2021). These bioinformatic tools implement novel algorithms that provide strain-level and quantitative profiling of microbiomes, as well as the ability to handle new features derived from the qRRS protocol that delivers high-fidelity base calls and yields that exceed Illumina’s maximum yield by as much as 50%. This new software is also backward compatible with amplicon sequencing and shotgun sequencing of metagenomes and meta-transcriptomes. These tools are being used to understand how multi-way plant-pathogen-microbe interactions modulate plant diseases and defense response pathways.
Other members of our group are involved in creating standards for reporting omics metadata (Dundore-Arias et al. 2020). This critical work will allow investigators to compare studies across the globe, thereby amplifying the effects of any individual study.
Microbiome and Plant Health and Disease
The microbiome topics most relevant to this project involve determining the relationships between microbiomes and plant health as well as between microbiomes and plant disease.
An example of this involves replant disease. Replant disease often occurs when certain crops are ‘‘replanted’’ in a soil that had previously supported the same or similar plant species. This disease typically leads to reductions in plant growth, crop yields, and production duration, and its etiology remains ill-defined. A study of replant disease from one of our members examined the relationships among the microbiome, replant disease severity, plant genotype, and seed meal applications (Wang and Mazzola, 2019). Here, the authors determined that (i) the rate of seed meal application was linked to replant disease severity, (ii) such applications were more effective with a specific plant genotype, and (iii) these beneficial effects were linked to microorganisms that make more anti-bacterial and anti-fungal compounds. This work provides a blueprint for other such studies, where several components of the phytobiome are examined to obtain a much more complete understanding of how all of these complex variables enable effective disease control.
Another example involves survivor trees. The Survivor Tree Phenotype is a natural disease tolerance phenomenon – where a small number of healthy citrus trees are found in orchards that are severely affected by Huanglongbing. To characterize this potentially valuable phenomenon, members of our group initiated a multiyear study to identify the microbes in these trees. The same trees in orchards from several geographical locations in Florida were annually assessed for their HLB disease severity ratings and their composition of microbes. This work identified trees with little to no decline in their health, providing evidence that the Survivor Tree Phenotype is a reproducible phenomenon. A small number of microbes were positively associated with this phenotype, suggesting that host-microbe interactions may be causing this phenotype (Ginnan et al. 2020). Towards the goal of creating an Huanglongbing management strategy, future work will involve testing these microbes for their ability to control disease.
Another example is the use of anaerobic soil disinfestation. Management of soilborne diseases in strawberries and other diseases used to be accomplished via methyl bromide fumigation. Because of environmental concerns, methyl bromide and other fumigants have been or are being phased out, creating a need for alternative disease management strategies. One of our members has performed a series of studies examining the use of anaerobic soil disinfestation as an alternative strategy to manage these diseases. In one of these studies, the efficacy of such treatments was linked to changes in the soil microbiomes (Mazzola et al. 2018). This work provides another important example of how the microbiome is important for disease management of soilborne plant pathogens. Additional research on this topic determined how soil metabolites were influenced by anaerobic soil disinfestation (Hewavitharana et al. 2019), providing key clues about the putative mechanisms driving this beneficial effect, and identifying molecules that may be used in future disease management strategies.
Cultural and Chemical Inputs and the Microbiome
Members of our group have also published several landmark papers on how cultural and chemical inputs affect the wheat microbiome. We were the first to document, with next-generation sequencing, that glyphosate applied at rates used by farmers, has minimal impact on both bacterial and fungal communities in the rhizosphere and bulk soil. (Schlatter et al. 2017a, 2018a, b). Biosolids from treated sewage are applied to fields in eastern Washington as a source of N. We showed that biosolids can shift both bacterial and fungal communities, even four years after the application (Schlatter et al. 2017b, 2019a). We also documented the bacterial and fungal microbiome of dust emitted from these biosolid treated fields (Schlatter et al. 2018c).
Many of the soils in eastern Washington are becoming acidified because of use of ammonia fertilizers over 60 years, and growers are adding lime to increase soil pH. In a series of papers, we examined how liming affects bacterial and fungal microbiomes (Schroeder et al. 2018, Yin et al. 2021). In a series of papers comparing long-term no-till sites to conventionally- tilled sites, we found that tillage had a stronger effect on fungal communities than bacterial communities (Yin et al. 2017, Poudyal-Sharma et al. 2017). Earthworms also play a major role in no-till systems, by moving carbon into deep soil profiles. We identified specific bacterial communities present in the drilosphere, lining the burrows of the earthworms, and also unique communities present in the gut and casts (Schlatter et al. 2019b, c). Soils in the hilly Palouse region of Eastern Washington are very deep wind deposited loess soils, with topsoil up to 10 ft deep. In our Mediterranean climate with little summer precipitation, wheat plants rely on moisture stored in this deep profile. We showed very distinct communities at each depth in a no-till system (Schlatter et al. 2020a). The top layer was enriched with copiotrophic bacteria that colonized roots and degraded above ground biomass. At 10 cm, there was an acidified layer with a distinct community of acid tolerant bacteria, with reduced richness and tolerance. In the lower layers were oligotrophic slow growing bacteria, and many more unknown taxa. Finally, we defined the core microbiome of wheat grown across 4 locations in distinct precipitation zones of Eastern Washington (Schlatter et al. 2018d, 2020b). There was tremendous variation in communities in the bulk soil of each location, but the rhizosphere selected for a narrower group of common taxa.
We have described how several different management practices associated with potato production in the Pacific Northwest influence the soil and potato microbiome. For example, we have described the short-term response of the soil microbial community to 1,3-dichlropropene application and, using amplicon sequencing to assess the microbial community, found minimal detectable response (Zeng et al. 2019). Additionally, we documented legacy effects of metam sodium use on the soil microbiome and how previous exposure to metam sodium changes the response of the microbial community if re- exposed to the fumigant (Li et al. 2022). We characterized the microbiome in soils closely associated with seed potato (tare soils) and found that the microbiome varies as a function of seed source and that a large number of potentially plant pathogenic taxa were detected by sequencing tare soil, including pathogens of plants other than potato (Delventhal et al. 2022a). Additionally, we found that the potato rhizosphere microbiome establishment appears to be heavily influenced by the microbial community of the soil in which the seed tuber is planted (Delventhal et al. 2022b). This suggests that on-farm management practices that influence the soil microbiome are an important contributing factor to rhizosphere microbiome establishment that could influence plant productivity.
Identification and Testing of New Biocontrol Agents
As mentioned in previous sections, the field of biological control of soilborne pathogens continues to expand (Lahlali et al. 2022). As of 2022, there are 29 Trichoderma products registered with EPA, over 30 with Pseudomonas, and 20 with Bacillus (http://npic.orst.edu/NPRO/). There are 13 bacterial based BCAs registered in the EU (Bonterra et al. 2022). But this list is expanding by discoveries in the microbiome, which is becoming a tool for finding biocontrol agents (Malacrino et al. 2022, Berg et al. 2021). For the first time, we can see all of the fungal and bacterial community members. In fact, although these BCAs have been extensively researched the last 40 years, they are often just one minor component of the total community. This is why the component of microbiome and phytobiome are of increasing importance in this project.
W-4147 members have been instrumental in discovering and testing many of these new biocontrol agents in the last five years (states doing the research in parentheses). These include:
Burkholderia ambifara, Bacillus simplex, B. safensis, Paenibacillus graminis and Lysobacter enzymogenes (NE) to control Pythium spp. and Rhizoctonia spp.
Trichoderma atroviride (AK)
Hyalorbilia aff. oviparasitica (basionym: Dactylella aff. oviparasitica) CA- to control Heterodera schachtii
Bacillus subtilis for alfalfa pathogens (DE)
Trichoderma spp. to suppress greenhouse diseases (Rhizoctonia and Pythium (NH)
Burkholderia spp. and Pseudomonas protegens for boxwood blight (VA)
Another exciting finding from the group (CA) is the discovery, characterization and application of bacteria belonging to the genus Collimonas, their antifungal properties, and their ability to work synergistically with Bacillus bacteria to protect plants from soilborne fungal pathogens (Doan et al, 2020). They discovered a novel Collimonas-produced antifungal metabolite (carenaemin) (Akum et al 2021), and found that fungi respond to and adapt after repeated exposure to Collimonas biocontrol bacteria (Mosquera et al, 2021). They are collaborating with UC Davis engineers to encapsulate and stabilize Collimonas bacteria for field application (Kawakita et al 2021a, Kawakita et al 2021b).
Members continue to look at the mode of action of BCAs, to gain insight on how they may work and be optimized.
This includes:
Pseudomonas spp, which produce antifungal compounds such as phenazine and 2,4-diacetylphloroglucol (WA) (Kwak and Weller 2013)
The role of biofilms and electron transport (redox potential and Fe availability) in colonization of roots by phenazine producers (WA) (LeTourneau et al. 2019)
The role of ribosomal proteins surfactin and plipastatin in biofilm formation (DE)
Bradyrhizobium japonicum, a PGPR, increases root biomass via regulation of auxin efflux transporters PIN2, PIN3, PIN7 and ABCB 19 (CA).
The role of signal molecules in zoosporic Phytophthora (Phytophthora erythroseptica) and how this may influence disease (ME) (Jiang et al. 2019)
The role of antifungal compounds in Lysobacter enzymogenes (NE) (Wang et al. 2015).
Disease Suppressive Soils and Plant Protecting Microorganisms
Over the last 20 years there have been surprising and exciting discoveries for natural methods to suppress or eliminate pathogens, and/or protect plants. One of these are naturally suppressive soils, which is a different paradigm than inoculating with BCAs. Disease-suppressive soils occur when indigenous microbes protect the plant against pathogens. Often the disease becomes established in a field, and causes disease for a few years, but then declines, even though the pathogen is still present. Understanding suppression is key to managing the agroecosystem to promote this phenomenon, which is sustainable long- term, and does not require any inputs. This is especially valuable for low-input systems or for organic agriculture.
Intensive studies of disease suppressive soils have led to the development of new methods of analysis (Gross et al. 2007, Borneman and Becker, 2007, Bolwerk et al. 2005, Benitez et al. 2007) and new insights into the nature of soilborne disease suppression (Weller et al. 2002, Hoitink and Boehm 1999). The most interesting direction has been the use of microbiome research to describe these complex communities. Members of W-4147 are recognized as leaders in this area, as evidenced by an invited review article titled “Disease Suppressive Soils: New Insights from the Soil Microbiome” which was published in Phytopathology in 2017 (Schlatter et al. 2017c). In this paper, Schlatter, Weller, Thomashow and Kinkel, all members of W- 4147, speculate on the future of research in this area, show three case studies (take-all of wheat (Gaeumannomyces graminis), Rhizoctonia bare patch (Rhizoctonia solani), and Streptomycetes spp.) and construct a series of testable hypotheses. Many of the advances in the study of suppressive soils have been made by members of W-4147.
These include:
Rhizoctonia root rot and bare patch (Yin et al. 2013, 2021)
Heterodera schachtii (Chen et al. 2021, Witte et al. 2021)
Take-all of wheat (Yang et al, 2022, submitted)
Apple replant (Cohen et al., 2005)
Methods that transform resident microbial communities in a manner which induces natural soil disease suppression have potential as components of environmentally sustainable systems for management of soilborne plant pathogens to reduce the need for pesticides.
Differentiation from other regional workgroups in the area of plant pathology
At the present time, there is no Multistate Project that completely overlaps with this group. The closest is S1083: Ecological and genetic diversity of soilborne pathogens and indigenous microflora. This project mainly focuses on crops in the Southern US, while ours focuses on cropping systems in the Western US. There are several important differences between the two regions, the main one being that water is more limited in the West, and there are more irrigated crops. Crops in the South are primarily centered on corn, soybean and peanuts, whereas there is a greater diversity of crops in the West, especially in California and Washington.
The rest of the Multi-State projects on plant disease focus on specific pathogens or cropping systems.
Objectives
-
Biocontrol via one or a small number of microbes and/or their products
-
Biocontrol via microbial communities and soil health
-
Creating and testing disease management and/or soil health strategies
-
Extension and outreach
Methods
We have a wide range of diverse research interests and programs in W-5157. But many of these are collaborative and cut across different states that focus on some important cropping systems. We present them according to objectives. Some of the collaborative themes include: potato soil health (WA, OR, ME), plant pathogenic nematodes (CA, WA, ME, MS), camelina biofuels (WA, CA and MT), soybean health (especially charcoal rot) (KS, MS) and enhancing efficacy of biopesticides (NH KS and others).The phytobiome and testing of biocontrol agents are other topics that are being researched by most of the members.
Objective 1. Biocontrol via one or a small number of microbes and/or their products
Coordinated Agricultural Project 1 on Camelina and Biofuels. Bacteria to Promote Growth of Camelina.
Members in WA, CA and MT are collaborating on a large Department of Energy CAP grant to increase the nitrogen use efficiency of camelina, a potential biofuels crop. They are isolating bacteria from the rhizosphere, and testing for plant growth promotion. Collaborators in WA have developed a collection of over 3000 bacterial isolates and are screening with organic and inorganic N sources. We are also screening over 200 lines in the field in MT and WA to identify lines that can give the maximum yield with minimum N inputs. Researchers in MT are screening another collection of bacteria from MT for plant growth promotion, using an agar-based screening method.
Coordinated Agricultural Project 2 on Cyst Nematode Management.
We are initiating a group project on cyst nematode management. Cyst nematodes of the family Heteroderidae are one of the most damaging groups of obligate pathogens of economically important crops worldwide (Miller 1986, Jones et al. 2013). The idea for this project came from the following important finding. We performed a study examining indigenous populations of a fungus in field soils cropped to sugar beets in the Imperial Valley of California. This fungus was formerly called Dactylella oviparasitica, but it now belongs to a group of nematophagous fungi called the Hyalorbilia oviparasitica clade (Yang et al. 2012, Chen et al. 2021, Witte et al. 2021, Smith Becker et al. 2022). We determined that soils with detectable levels of these fungi all produced similar and substantial reductions in the population densities of the sugarbeet cyst nematode (Heterodera schachtii) after two nematode generations, all below the damage threshold. This fungus has also been shown to be equally effective in different soil types (Olatinwo et al. 2006). The component of this project that is relevant to Objective 1 is to determine why this fungus is effective in different soil types, because this could have broad implications for all soil-borne biological control. Project members will include Ole Becker, James, Borneman, Tim Paulitz, Gretchen Sassenrath, and Tessie Wilkerson.
Coordinated Agricultural Project 3: Enhancing efficacy of biopesticides:
Research has demonstrated that different soil types, plant species and even cultivars harbor unique microbial communities (Parnell et al., 2016). Little is known however, about how these variables affect establishment, function, and population dynamics of biocontrol microbes. For example, soil physical and chemical properties can influence microbial activity and may influence biopesticide efficacy as research has shown that a biocontrol agent can suppress disease in one soil/substrate and not another (Hoitink and Boehm, 1999). In this project we propose to study effects of soil physiochemical properties on biopesticide efficacy for suppression of soilborne diseases. Projects from group members in ME, NH, WA, CA, KS will study these effects in soil-based outdoor production systems and soilless (peat and wood fiber based) substrates in greenhouse production systems. We also propose to identify host and regionally adapted novel biocontrol agents to obtain next generation microbes that are effective in the control of major diseases using potato as a model crop.
Objective 2. Biocontrol via microbial communities and soil health
Coordinated Agricultural Project 1 on Camelina Biofuels. As part of a Department of Energy CAP grant, researchers in CA, MT and WA are describing the microbiome of camelina, with the goal of identifying communities that benefit the plant through growth promotion, disease suppression and drought tolerance. They will sample soils across 33 locations in eastern Washington and do amplicon sequencing for 16S and ITS to quantify bacteria and fungi. They will test 200 individual camelina lines in the field under high and low nitrogen conditions, to identify those that have the highest Nitrogen Use Efficiency (NUE) and those that can provide sustainable yield even under low N conditions. They will also do metagenomics shotgun sequencing the JGI, based on plants growing in high and low N, to identify the role microbes may plan in nutrient update. Finally, we will do metabolomics with the bacterial strains, a collaboration with researchers at DOE Lawrence Livermore Labs.
Coordinated Agricultural Project 4- Soil Health in Potato Production. Much of the active research in soil health is conducted in grain cropping systems, which differ greatly from potato cropping systems. Thus, soil health in potato cropping systems is poorly defined and it is unclear which indicators are most important to assess soil health as it relates to potato productivity. Although soilborne plant pathogenic microorganisms have not traditionally been considered in the context of soil health assessments, they are extremely important in potato cropping systems and their management has broad-scale consequences to soil health in potato systems. As part of a USDA SCRI CAP grant, members of W-5147 from Maine, Oregon and Washington have established potato rotation experiments to characterize how soil microbiome diversity and structure varies as a function of agricultural practices including, crop rotation, use of cover crops, soil amendments, and pesticide applications. W-5147 members from these states will continue to use different agricultural practices to experimentally manipulate soil microbial composition and physiochemical properties and collect data to determine how agricultural practices affect soil microbiomes, crop health and yield. From the rotation experiments, a full panel of soil measurements will be taken each year, including soil physical, chemical, and biological properties. Additionally, metagenomics sequencing will be used to describe soil microbiome functional capability and learn if the diverged microbiomes are functionally similar across states. We plan to compare metagenomes between bulk and rhizosphere soils to learn if core microbial communities recruited by potato result in community structures and taxa that are more similar across states when compared to bulk soils. Finally, understanding the function of the microbial communities within the soil and how it relates to nutrient availability may further explain disease incidence within a field and can be further linked to good vs. poor preforming sites and/or soil characteristics. The primary goal of this research is to identify agricultural practices that optimize soil microbiome function and soil characteristics to improve potato yield and quality.
Objective 3. Creating and testing disease management and/or soil health strategies
Coordinated Agricultural Project 2 on Cyst Nematode Management. We are initiating a group project on cyst nematode management, see Objective 1 for more information. We have shown that the degree of nematode suppression was not correlated to the initial population densities of these fungi (Smith Becker et al. 2022). To our knowledge, this is the first study (Smith Becker et al. 2022) showing that the presence of an indigenous biological control microorganism could be used to accurately predict whether the population densities of a plant pathogen would be suppressed. One logical new direction is to determine whether we will obtain similar results when some key variables are changed, such as geographic region, crop species, soil type, and/or the cyst nematode population. If we obtain the expected results, we anticipate that we will be able to translate this knowledge into cyst nematode management strategies that enable us to predict which soils can be safely planted with cyst-nematode susceptible crops. Several members of our multistate project will contribute to this work – to develop strategies to manage the sugarbeet cyst nematode, the soybean cyst nematode, and the cereal cyst nematode. Project members will include Ole Becker, James, Borneman, Tim Paulitz, Gretchen Sassenrath, and Tessie Wilkerson.
Coordinated Agricultural Project 5 Measuring the impact of soil health on soybean disease (KS and MS)
There is uncertainty in how soil management or “soil health” parameters relate to disease suppression (Pimentel et al., 2022). The Soil Management Assessment Framework (SMAF) has identified five factors that are indicative of soil microbial activity (Andrews et al., 2004). SMAF has been adopted by the NRCS as an indicator of soil health (Stott, 2019). These factors include: organic carbon, nitrogen, active carbon, wet aggregate stability and soil respiration. These give an indication of the activity of the soil microbiome, though they do not measure the microbes directly. Total microbial biomass and the ratio of fungi to bacteria have also been shown to be related to disease suppression in soils (Janvier et al., 2007). Although several different metrics of soil health have been developed (CASH, Haney, PLFA, etc.), there is no clear indication of how these metrics relate to either the productive capacity ($/acre) or the disease abundance (CFU of disease organisms relative to beneficial microbes) (Janvier et al., 2007). Our goal is to develop an understanding of the “health” of a soil from the standpoint of productivity ($/ac) and disease suppression (lower CFUs of disease-causing organisms). To this end, we have chosen key metrics that are important for growth of microbes. We will focus on quantitating these key factors, and developing relationships between these factors and disease prevalence (CFUs), using Macrophomina phaseolina as the model. M. phaseolina is a soil-borne fungus responsible for charcoal rot disease in many crops, including soybeans (Mengistu et al., 2011; Perumal et al., 2020), sorghum (Adeyanju et al., 2015), and strawberries.
Management practices to alter the soil community structure will be implemented in replicated research plots. Treatments will include management practices that are likely to increase disease (e.g. soybeans and corn stubble, both hosts of disease organisms), treatments that are likely to decrease disease (e.g. brassica cover crop (Sassenrath et al., 2017, 2019), animal manure, and solarization), and a fallow control. Soil samples will be taken prior to implementing treatments, at multiple intervals during the growing season, and after harvest of the cash crop to determine: soil microbial community structure, pathogen populations, and soil nutrient status. Final crop yield will be measured. Standard soil nutrient analysis will be performed to measure changes in pH, total organic matter, N, P, K, total organic carbon, total N, NO3-N, NH4-N, along with key micronutrients S, Ca, Mg, and Na. Wet aggregate stability, soil respiration, total soil microbial biomass, and fungal:bacterial ratio will be measured.
In addition, we will quantitate the disease presence of Macrophomina phaseolina in soil and plants at three time points during the growing season (prior to planting, mid-season, and at harvest) by measuring CFUs. M. phaseolina populations will be determined by counting the number of colony-forming units (CFUs). Charcoal rot disease severity will be measured by randomly selecting ten plants per plot at the R7-R8 growth stage for root and stem severity ratings. The plants will be scored by splitting the stem and taproot of each plant and rating the degree of gray discoloration and microsclerotia in the vascular and cortical tissues on a scale from 1 to 5. M. phaseolina populations in roots and soil will be estimated by grinding the samples. The ground plant tissue and soil samples will be plated on microbiological media and incubated. CFUs of M. phaseolina will be counted and transformed to CFUs per gram of root tissue or gram of soil. To examine other potential disease organisms, we will work to develop PCR tests with disease-specific primers. This test gives a qualitative measure of disease presence, and, in addition to Macrophomina, will allow us to detect Fusarium, Phytophthora, Phomopsis, and Pythium, all common soil-borne diseases that substantially reduce crop yield and quality. We will also assess Sudden Death Syndrome (SDS) and soybean cyst nematode populations, which are major problems in soybean production.
Objective 4. Extension and outreach. SEE SECTION ON OUTREACH PLAN IN THE OUTREACH SECTION
MILESTONES (see below or in the Attachments section)
Projects, Activities, and Milestones | Personnel (State) |
Year |
|||||
1 |
2 |
3 |
4 |
5 |
|||
CAP 1 –Camelina Biofuels | |||||||
X | |||||||
Make rhizosphere collection of bacteria | x | ||||||
Sequence rhizosphere microbiome with amplicon sequencing | x | X | |||||
Complete metagenomic analysis of rhizosphere bacteria grown with 10 camelina lines grown under low and high N conditions | Timothy Paulitz (WA), Jed Eberly (MT), Susanne Tringe (CA), Qing Yan (MT), Elle Barnes (CA), Hao Peng (CA) | x | X | ||||
Complete metabolomics study on selected bacteria from collection | X | ||||||
Complete field analysis of camelina lines to select high and low NUE lines | x | x | |||||
CAP 2 – Cyst Nematode Management | |||||||
Initiate studies to obtain preliminary results for grants | Ole Becker (CA), James, Borneman (CA), Tim Paulitz (WA), Gretchen Sassenrath (KS), and Tessie Wilkerson (MS) | X | |||||
Complete studies to obtain preliminary results for grants | X | ||||||
Write and submit grant proposals | X | ||||||
Initiate studies for funded projects | X | ||||||
Continue studies for funded projects | X | ||||||
CAP 3 – Enhancing efficacy of biopesticides: | |||||||
Conduct preliminary experiments in growth room studies to survey soils and substrates for biocontrol agent survival and disease suppression, measure soil physiochemical properties | Anissa Poleatewich (NH), Gretchen Sassenrath (KS), Johan Leveau (CA), Jay Hao (ME), Ken Frost (OR), Tim Paulitz, (WA) | x | x | ||||
Collect and screen biocontrol isolates. Identify top performing biocontrol isolates | x | x | |||||
Complete greenhouse and field studies. Analyze data to identify effects of substrate properties on biocontrol agent efficacy. | x | x | x | ||||
Develop extension materials; deliver to farmers and industry through field days, popular press, and media. Prepare scientific publications | x | ||||||
CAP 4 Soil Health in Potato Production. | |||||||
Coordination of sampling protocols and treatments | Timothy Paulitz (WA), Jay Hao (ME), Ken Frost (OR) | X | |||||
Conduct field experiments (3 sites, 12 treatments) | X | X | X | X | X | ||
Sample bulk and rhizosphere soils for assessment | X | X | X | X | X | ||
Describe relationships between agricultural practices, soil health indicators, crop health, yield and quality | X | X | X | ||||
Identify redundant soil health indicators | X | X | X | ||||
Identify soil health indicators linked to greater microbial community diversity, healthier plants and higher yields | X | X | |||||
Results fed into extension and outreach activities (Obj. 4) | X | X | X | X | X | ||
CAP 5. Measuring the impact of soil health on soybean disease (KS and MS) | |||||||
Implement test plots in research fields; collect soil samples from test plots and production farms | Chris Little (KS), Gretchen Sassenrath (KS), Tessie Wilkerson (MS), Tim Paulitz (WA), | x | x | x | x | ||
Assess soil health factors and microbial activity | x | x | x | x | |||
Measure abundance of disease organisms | x | x | x | ||||
Develop relationships between soil health factors, microbial activity, and disease prevalence | x | x | x | ||||
Develop educational programs and materials; deliver to farmers and industry through field days, popular press, and media | x | x | x | x |
Measurement of Progress and Results
Outputs
- Refereed publications, abstracts and meeting proceedings.
- Extension bulletins
- Transmission of technology to stakeholders via field days, webinars and workshops.
- Training of qualified personnel as undergraduate and graduate students and postdoctoral researchers.
- Best management practices for disease control
- Improved diagnostics and detection of soilborne pathogens
- Recommendations and testing of biocontrol agents under greenhouse and field conditions.
- Recommendations for cultural practices and rotations to enhance soil health and disease suppression.
Outcomes or Projected Impacts
- Outcome 1. Knowledge and understanding of the phytobiome of agricultural systems and the benefits and services they provide to crop productivity
- Outcome 2. Understanding of not only the identity of the key drivers providing ecosystem services, but also their function through the use of metagenomics, transcriptomics and metabolomics.
- Outcome 3. Identification of communities and consortia involved in natural suppression of soilborne plant pathogens
- Outcome 4. Reduced use of chemical pesticides and increased use of biologically based products.
- Outcome 5. Reduced damage by soilborne pathogens and increased crop productivity and profitability.
- Outcome 6. Safe, low-cost agricultural products
- Outcome 7. Benefits to growers, consumers, and the environment by making significant progress in producing low cost safe agricultural products.
- Outcome 8. A greater understanding of the basic molecular and biochemical mechanisms will allow a better selection and improvement of existing new strains, and a more rational implementation of these organisms.
- Outcome 9. Knowledge of the genomic and biochemical diversity of microbial communities and biocontrol agents, and how they function in agroecosystems.
- Outcome 10. Understanding how the biocontrol agents interact with the plant and the environment, to predict their limitations and inconsistency in the field.
- Outcome 11. Expanded tool kit of disease management options for both organic and conventional growers, leading to improved agricultural productivity and sustainability.
Milestones
(0):The Milestones Supplemental Table can be view at the end of the Methods section or by loading up the attachment.Projected Participation
View Appendix E: ParticipationOutreach Plan
The goal of the Outreach Plan for the W-5147 Project will be to deliver fundamental and applied information about biological control of soilborne pathogens. We will target growers, other members of the agricultural community, scientists, and the general public using several mechanisms.
- Outreach to Growers. Over 50% of our members have extension appointments and regularly meet, consult, and teach growers, pest control advisors, and industry representatives via field days and workshops. Over the course of the last 5 years, 344 talks and extension bulletins were presented.
- Online Outreach. Members of group engage in numerous forms of online media - e.g., our members created the Cornell Soil Health Website (http://soilhealth.cals.cornell.edu/). Growers can send in samples to have soil health status evaluated and to learn of major soil quality constraints that need to be addressed.
- Outreach to Industry. Many of the products developed by our group are being marketed by the biocontrol industry including products such as Root Shield and Plant Shield.
- Training the Next Generation of Biocontrol Scientists and Practitioners. Almost all of our members have teaching responsibilities. They teach courses in biological control and plant pathology, and train graduate and undergraduate student researchers.
- Public education to general community and grades K-12. Our members participate in science fair judging, Master Gardener Training, 4-H and others.
- Providing Information to Policy Makers. Our members will continue to be engaged with policy makers at all levels - e.g., see Subcommittee in Governance section.
- Publishing Results in Peer-Reviewed Scientific Journals. Our group has an excellent publication record, which we plan to continue. In the last 5 years, we have published 362 peer reviewed publications, 23 book chapters, 3 theses and 66 conference proceedings/abstracts by our members, among many other activities.
Organization/Governance
Governance of the W-5147 Project will follow recommended guidelines with the Technical Committee being comprised of research scientists, extension specialists, an Administrative Advisor, and a NIFA Representative. Our Administrative Advisor will be Scot Hulbert. Officers of the Technical Committee will be the Chair and Secretary. The Secretary will be elected each year and will advance to Chair the following year. The location and organizers of the Annual Meetings will be determined by an election. At the Annual Meetings, presentations will be made describing research and extension accomplishments by the members of the Technical Committee. The Secretary will be responsible for taking the minutes at the Annual Meetings, and will work with the Chair to create the Annual Report. Consistent with the sustainability goals of the USDA and the Objectives of the W-5147 Project, we will form a subcommittee that will endeavor to increase funding for soilborne biological control research.
We note that USDA used to have a program that only funded biological control research. One of the goals of the W-5147 Project will be to resurrect that program or one that has similar goals. These goals will include increasing the safety and sustainability of US Agriculture through fundamental and applied research and extension projects in biological control. Finally, several new members have been recruited into the project since the last renewal including Johan Leveau, Rachael Vanette, Harsh Bais, Chris Little, Gretchen Sassenrath, Anissa Poleatewich, Bode Olukolu, Kiran Mysore, Chuanxue Hong, Ken Frost, Jed Eberly, Ryan Graebner and Mike Kolomiets, all who have participated in meetings and reports.
Literature Cited
Adeyanju, A, Little, C, Yu, J, Tesso, T. 2015. Genome-wide association study on resistance to stalk rot diseases in grain sorghum. G3 Genes/Genomes/Genetics. 5(6):1165-1175. https://doi.org/10.1534/g3.114.016394.
Akum, FN, Kumar, R, Lai, G, Williams, CH. Doan, HK, Leveau, JHJ. 2021. Identification of Collimonas gene loci involved in the biosynthesis of a diffusible secondary metabolite with broad-spectrum antifungal activity and plant-protective properties. Microbial Biotechnology 14: 1367-1384. https://doi.org/10.1111/1751-7915.13716.
Andrews, S.S., Karlen, D.L., Cambardella, C.A. 2004. The soil management assessment framework: A quantitative soil quality evaluation method. SSSA, 68:1945-1962.
Batista, BD., Singh, BK. 2021. Realities and hopes in the application of microbial tools in agriculture. Microbial Biotechnology 14:1258-1268. https://doi.org/10.1111/1751-7915.13866.
Benitez, MS, Tustas, FB, Rotenberg, D, Kleinhenz, MD, Cardina, J, Stinner, D, Miller, SA, McSpadden Gardener, BB. 2007. Multiple statistical approaches of community fingerprint data reveal bacterial populations associated with general disease suppression arising from the application of different organic field management strategies. Soil Biology & Biochemistry 39: 2289-230.
Berg, G, Kusstatscher, P, Abdelfattah, A, Cernava, T, Smalla, K. 2021. Microbiome modulation—toward a better understanding of plant microbiome response to microbial inoculants. Front. Microbiol. 12, 650610 https://doi.org/10.3389/fmicb.2021.650610.
Biological Pesticide Products Industry Alliance. 2023. Markets for Biological Products: Global Market Landscape. https://www.bpia.org/markets-for-biological-products-global-market-landscape/.
Bolwerk, A, Lagopodi, AL, Lugtenberg, BJJ, Bloemberg, GV. 2005. Visualization of interactions between a pathogenic and a beneficial Fusarium strain during biocontrol of tomato foot and root rot. Molecular Plant-Microbe Interactions 18: 710-721.
Borneman, J, Skroch, PW, Jansen, JA, O'Sullivan, KL, Palus, JA, Rumjanek, NG, Nienhuis, J, Triplett, EW. 1996. Molecular microbial diversity of an agricultural soil in Wisconsin. Applied and Environmental Microbiology 62:1935-1943.
Borneman, J, Triplett, EW. 1997. Molecular microbial diversity in eastern Amazonian soils: Evidence for unusual microorganisms and population shifts caused by deforestation. Appl Environ Microbiol 63:2647-53.
Borneman, J. 1999. Culture-independent identification of microorganisms that respond to specified stimuli. Applied and Environmental Microbiology 65:3398-3400.
Borneman, J, Becker, JO. 2007. Identifying microorganisms involved in specific pathogen suppression in soil. Annual Review of Phytopathology 45: 153-172.
Canfora, L, Costa, C, Pallottino, F, Mocali, S. 2021. Trends in soil microbial inoculants research: A science mapping approach to unravel strengths and weaknesses of their application. Agriculture 11:158. https://doi.org/10.3390/agriculture11020158.
Chaloner, TM, Gurr, SJ, Bebber, DP. 2021. Plant pathogen infection risk tracks global crop yields under climate change. Nature Climate Change 11:710–715. https://doi.org/10.1038/s41558-021-01104-8.
Chen Y-Y, Koike ST, Logan GD, Drozd C, De Oliveira Silva J, Colindres NB, Peacock BB, Smith Becker J, Loffredo A, Wu H, Ruegger PM, Becker JO, Borneman J. 2021. Detection of Nematophagous fungi from Heterodera schachtii females using a baiting experiment with soils cropped to Brassica species from California’s Central Coast. PhytoFrontiers 1:1-9 https://doi.org/10.1094/PHYTOFR-07-20-0009-R.
Cohen, MF, Yamasaki, H, Mazzola, M. 2005. Brassica napus seed meal soil amendment modifies microbial community structure, nitric oxide production, and incidence of rhizoctonia root rot. Journal of Soil Biology and Biochemistry. 37:1215-1227.
Delgado-Baquerizo, M, Guerra, CA, Cano-Díaz, C, Egidi, E, Wang, JT, Eisenhauer, N, Singh, BK, Maestre. FT. 2020. The proportion of soil-borne pathogens increases with warming at the global scale. Nature Climate Change 10:550–554. https://doi.org/10.1038/s41558-020-0759-3.
Delventhal, K, Skillman, V, Li, X, Busby, P, Frost, KE. 2022a. Characterizing variation in the bacterial and fungal tare soil microbiome of seed potato. Phytobiomes https://doi.org/10.1094/PBIOMES-11-22-0092-R.
Delventhal, K, Busby, P, Frost, KE. 2022b. Tare soil alters the composition of the developing potato rhizosphere microbiome. Phytobiomes https://doi.org/10.1094/PBIOMES-11-22-0093-R.
Doan, HK, Maharaj, NN, Kelly, KN, Miyao, EM, Davis, M, Leveau, JH.J. 2020. Antimycotal activity of Collimonas isolates and synergy-based biological control of Fusarium wilt of tomato. Phytobiomes Journal 4:64-74. https://doi.org/10.1094/PBIOMES-05-19-0027-R.
Dundore-Arias, JP, Eloe-Fadrosh, EA, Schriml, L.M., Beattie, G.A., Brennan, F.P., Busby, P.E., Calderon, R.B., Castle, S.C., Emerson, J.B., Everhart, S.E., Eversole, K., Frost, K.E., Herr, J.R., Huerta, A.I., Iyer-Pascuzzi, A.K., Kalil, A.K., Leach, J.E., Leonard, J., Maul, J.E., Prithiviraj, B., Portrykus, M. Redekar, N.R., Rojas, J.A., Silverstein, K.A.T., Tomso, D.J., Tringe, S.G., Vinatzer, B.A., and Kinkel L.L. 2020. Community-driven metadata standards for agricultural microbiome research. Phytobiomes. 4(2):115-121. https://doi.org/10.1094/PBIOMES-09-19-0051-P.
Environmental Production Agency. 2017. Biopesticide 2017 Workplan. https://www.epa.gov/pesticide-registration/biopesticide-2017-workplan.
Evin, B, Frost, K, Kinkel, L, MacGuidwin, A, Knuteson, D, Gevens, A, Larkin, R. 2021. Disease suppressive soils. USDA NIFA Enhancing Soil Health in U.S. Potato Production Systems Extension Publication.
Garcia-Aroca, T, Price, PP, Tomaso-Peterson, M, Allen, TW, Wilkerson, TH., Spurlock, TN, Faske, TR, Bluhm, B, Conner, K, Sikora, E, Guyer, R, Kelly, H, Squiers, BM, Doyle, VP. 2021. Xylaria necrophora, sp. nov., is an emerging root-associated pathogen responsible for taproot decline of soybean in the southern United States. Mycologia, 113:2, 326-347. https://doi.org/10.1080/00275514.2020.1846965.
Ginnan NA, Dang T, Bodaghi S, Ruegger PM, McCollum G, England G, Vidalakis G, Borneman J, Rolshausen PE, Roper MC. 2020. Disease-induced microbial shifts in citrus indicate microbiome-derived responses to Huanglongbing across the disease severity spectrum. Phytobiomes 4:375-387. https://doi.org/10.1094/PBIOMES-04-20-0027-R.
Gross, H, Stockwell, VO, Henkels, MD, Nowak-Thompson, B, Loper, JE, Gerwick, WH 2007. The genomisotopic approach: A systematic method to isolate products of orphan biosynthetic gene clusters. Chemistry & Biology (Cambridge) 14:53-63.
Hewavitharana, SS, Klarer, E, Reed, AJ, Leisso, R, Poirer, B, Honaas, L, Rudell, DR, Mazzola, M. 2019. Temporal dynamics of the soil metabolome and microbiome during simulated anaerobic soil disinfestation. Frontiers in Microbiology doi:10.3389/fmicb.2019.02365.
Hoitink, HAJ, Boehm, MJ. 1999. Biocontrol within the context of soil microbial communities: A substrate-dependent phenomenon. Annual Review of Phytopathology 37:427-446.
Janvier, C., Villeneuve, F., Alabouvette, C., Edel-Hermann, V., Mateille, T., Steinberg, C. 2007. Soil health through soil disease suppression: Which strategy from descriptors to indicators? Soil Biology and Biochemistry. 39:1-23.
Jenkins, D, Ory, J. 2016. 2016 National Organic Research Agenda. Organic Farming Research Foundation. https://ofrf.org/wp-content/uploads/2019/09/NORA_2016_final9_28.pdf?utm_source=OFRF+General+Mailing+List+1&utm_campaign=c62542ad21-Press+Release+Call+for+Abstracts_COPY_01&utm_medium=email&utm_term=0_4e39bea10a-c62542ad21-22598513.
Jiang, H, Hwang, HW, Ge, T, Cole, B, Perkins, B, Hao, J. 2019. Leucine Regulates Zoosporic Germination and Infection by Phytophthora erythroseptica. Front. Microbiol. 10:00131. https://doi.org/10.3389/fmicb.2019.00131.
Jones, JT, Haegeman, A, Danchin, EGJ, Gaur, HS, Helder, J, Jones, MGK, Kikuchi, T, Manzanilla-Lopez, R, Palomares-Rius, JE, Wesemael, WML, Perry, RN. 2013. Top 10 plant-parasitic nematodes in molecular plant pathology. Molecular Plant Pathology 14(9):946-961.
Kawakita, R, Leveau, JHJ, Jeoh, T. 2021a. Comparing Fluidized Bed Spray-Coating and Spray-Drying Encapsulation of Non- Spore-Forming Gram-Negative Bacteria. Industrial Biotechnology 17:283-289. https://doi.org/10.1089/ind.2021.0019.
Kawakita, R, Leveau, JHJ, Jeoh, T. 2021b. Optimizing viability and yield and improving stability of Gram-negative, non-spore forming plant-beneficial bacteria encapsulated by spray-drying. Bioprocess Biosyst Eng 44:2289-2301. https://doi.org/10.1007/s00449-021-02604-9.
Kleczewski, NM, Bowman, ND. 2021. An Observation of Corn Tar Spot Dispersal from Agricultural Fields to an Isolated Urban Plot. Plant Health Progress 22:69–71. https://doi.org/10.1094/PHP-10-20-0082-BR.
Kuster, RD, Yencho, GC, Olukolu, BA. 2021. ngsComposer: an automated pipeline for empirically based NGS data quality filtering. Briefings in bioinformatics 22:bbab092.
Kwak, YS, Weller, DM. 2013. Take-all of wheat and natural disease suppression: A review. Plant Pathology Journal 29: 125-135.
Lahlali, R, Ezrari, S, Radouane, N, Kenfaoui, J, Esmaeel, Q, El Hamss, H, Belabess, Z, Ait Barka, E. 2022. Biological Control of Plant Pathogens: A Global Perspective. Microorganisms 10:596. https://doi.org/10.3390/microorganisms10030596.
Lazo, PL. 2023. Millions of global agricultural crops are lost to pests annually - FAO. Prensa Latina. https://www.plenglish.com/news/2023/05/12/millions-of-global-agricultural-crops-are-lost-to-pests-annually-fao/.
LeTourneau, MK, Marshall, MJ, Grant, M, Freeze, PM, Strawn, DG, Lai, B, Dohnalkova, AC, Harsh, JB, Weller, DM, Thomashow, LS. 2019. Phenazine-1-Carboxylic Acid-Producing Bacteria Enhance the Reactivity of Iron Minerals in Dryland and Irrigated Wheat Rhizospheres. Environ. Sci. Technol. 53:14273–14284. https://doi.org/10.1021/acs.est.9b03962.
Li, X, Skillman, V, Dung, J, Frost, KE. 2022. Legacy effect of fumigation on soil bacterial and fungal communities and their response to metam sodium application. Environmental Microbiome 79:59 https://doi.org/10.1186/s40793-022-00454-w.
Malacrino, A, Abdelfattah, A, Berg, G, Benitez, M-S, Bennett, AE, Bottner, L, Xu, S, Schena, L. 2022. Exploring microbiomes for plant disease management. Biological Control 169: 104890. https://doi.org/10.1016/j.biocontrol.2022.104890.
Mazzola, M, Muramoto, J, Shennan, C. 2018. Anaerobic disinfestation induced changes to the soil microbiome, disease incidence and strawberry fruit yields in California field trials. Applied Soil Ecology 127:74-86.
Marks, M, Ruark, M, Lankau, R, Kinkel, L, Frost, K. 2020a. What is the microbiome? USDA NIFA Enhancing Soil Health in U.S. Potato Production Systems Extension Publication. https://potatosoilhealth.cfans.umn.edu/education.
Marks, M, Ruark, M, Steinke, K, Frost, K. 2020b. What makes healthy soils? USDA NIFA Enhancing Soil Health in U.S. Potato Production Systems Extension Publication. https://potatosoilhealth.cfans.umn.edu/education.
Mengistu, A, Arelli, PA, Bond, JP, Shannon, GJ, Wrather, AJ, Rupe, JB, Chen, P, Little, CR, Canaday, CH, Newman, MA, Pantalone, VR. 2011. Evaluation of soybean genotypes for resistance to charcoal rot. Plant Health Progress. 12(1): https://doi.org/10.1094/PHP-2010-0926-01-RS.
Miller, LI. 1986. Economic Importance of Cyst Nematodes in North America. Cyst Nematodes. Nato ASI Series. Vol 121. F. Lamberti, Taylor, C.E. (eds). Boston, MA, Springer.
Mosquera, S, Leveau, JHJ, Stergiopoulos, I. 2021. Repeated Exposure of Aspergillus niger Spores to the Antifungal Bacterium Collimonas fungivorans Ter331 Selects for Delayed Spore Germination. Applied and Environmental Microbiology 87:00233-21. https://doi.org/10.1128/AEM.00233-21.
Mueller, DS, Wise, KA, Sisson, AJ, Allen, TW, Bergstrom, GC, et al. 2020. Corn Yield Loss Estimates Due to Diseases in the United States and Ontario, Canada, from 2016 to 2019. Plant Health Progress 21:238-247. https://doi.org/10.1094/PHP-05-20-0038-RS.
Muyzer G, de Waal EC, Uitterlinden AG. 1993. Profiling of complex microbial populations by denaturing gradient gel electrophoresis analysis of polymerase chain reaction-amplified genes coding for 16S rRNA. Applied & Environmental Microbiology 59:695-700.
National Agricultural Statistics Service. 2023. Crop Production. https://www.nass.usda.gov/.
Olatinwo, R, Becker, JO, Borneman, J. 2006. Suppression of Heterodera schachtii populations by Dactylella oviparasitica in four soils. Journal of Nematology. 38:345-8.
Olukolu BA, Yencho, GC. 2022. A quantitative Reduced Representation Sequencing (qRRS) of genomes; a paradigm shift in NGS-based genotyping. In: Merkle, S.A. and Levi, E.M. (Eds.) Proceedings of the 36th Southern Forest Tree Improvement Conference (SFTIC). https://taes.tennessee.edu/publication/show_pub.asp?pub=31325.
Panth, M, Hassler, SC, Baysal-Gurel, F. 2020. Methods for Management of Soilborne Diseases in Crop Production. Agriculture-Basel Volume 10, Article 16, https://doi.org/10.3390/agriculture10010016.
Parnell JJ, Berka R, Young HA, Sturino JM, Kang Y, Barnhart DM, DiLeo MV. 2016. From the Lab to the Farm: An Industrial Perspective of Plant Beneficial Microorganisms. Front Plant Sci. 7(August):1–12. https://doi.org/10.3389/fpls.2016.0111
Perumal, R, Tomar, SS, Bandara, A, Maduraimuthu, D, Tesso, TT, Prasad, PVV, Upadhyaya, HD, Little, CR. 2020. Variation in stalk rot resistance and physiological traits of sorghum genotypes in the field under high temperature. J. General Plant Pathology. 86:350-359. https://doi.org/10.1007/s10327-020-00940-4.
Pimentel, MF, Srour, AY, Warner, AJ, Bond, JP, Bradley, CA, Rupe, J, Chilvers, MI, Rojas, JA, Jacobs, JL, Little, CR, Robertson, AE, Giesler, LJ, Malvick, D, Wise, K, Tenuta, A, Fakhoury, AM. 2022. Ecology and diversity of culturable fungal species associated with soybean seedling diseases in the Midwestern United States. J. Applied Microbiology. 132:3797-3811. DOI: 10.1111/jam.15507.
Poudyal-Sharma, D, Schlatter, D, Yin, CT, Hulbert, S, Paulitz, TC. 2017. Long-term no-till: a major driver of fungal communities in dryland wheat cropping systems. PloS One 12: 10.1371/journal.pone.018611.
Research and Markets. 2018. Global Agricultural Microbials Markets 2022-2026. https://www.researchandmarkets.com/reports/5681488/global-agricultural-microbials-market-2022-2026#product--toc.
Ruegger, P, Clark, R, Weger, J, Braun, J, Borneman, J. 2014. Improved resolution of bacteria by high throughput sequence analysis of the rRNA internal transcribed spacer. J. Microbiol. Methods 105:82-87.
Sassenrath, GF, Little, CR, Hsiao, CJ, Shoup, DE, Lin, X. 2017. Cover crop system to control charcoal rot in soybeans. Kansas Agricultural Expt. Station Res. Reports. 3(2): https://doi.org/10.4148/2378-5977.1383.
Sassenrath, GF, Little, CR, Roozeboom, K, Lin, X, Jardine, D. 2019. Controlling soil-borne disease in soybean with a mustard cover crop. Kansas Agricultural Expt. Station Res. Reports. 5(2): https://doi.org/10.4148/2378-5977.7740.
Schlatter, DC, Yin, C, Hulbert, S, Burke, I, Paulitz, TC. 2017a. Impacts of repeated glyphosate use on wheat-associated bacteria are small and depend on glyphosate use history. Applied and Environmental Microbiology 83: DOI: 10.1128/AEM.01354-17.
Schlatter, DC, Schillinger, WF, Bary, AI, Sharratt, BS, Paulitz, TC. 2017b. Biosolids and conservation tillage: Impacts on soil fungal communities in dryland wheat-fallow cropping systems. Soil Biology and Biochemistry. 115:556-567.
Schlatter, D, Kinkel, L, Thomashow, L, Weller, D, Paulitz, T, 2017c. Disease Suppressive Soils: New insights from the soil microbiome. Phytopathology. 107:1284-1297. https://doi.org/10.1094/PHYTO-03-17-0111-RVW.
Schlatter, DC, Burke, I, Paulitz, TC. 2018a. Succession of fungal and oomycete communities in glyphosate-killed wheat roots. Phytopathology. 108:582-594.
Schlatter, DC, Yin, C, Burke, I, Hulbert, S, Paulitz, TC. 2018b. Location, root proximity, and glyphosate-use history modulate the effects of glyphosate on fungal community networks of wheat. Microbial Ecology https://doi.org/10.1007/s00248-017-1113-9.
Schlatter, DC, Schillinger, WF, Bary, AI, Sharratt, BS, Paulitz, TC. 2018c. Dust-associated microbiomes from dryland wheat fields differ with tillage practice and biosolids application. Atmospheric Environment. 185:29-40. 2018.
Schlatter, DC, Kahl, K, Carlson, BR, Huggins, DR, Paulitz, TC. 2018d. Fungal community composition and diversity vary with soil depths and landscape position in a no-till wheat cropping system. FEMS Microbiology Ecology. https://doi.org/10.1093/femsec/fiy098.
Schlatter, DC, Paul, NC, Shah, DH, Schillinger, WF, Bary, AL, Sharratt, BS, Paulitz, TC. 2019a. Biosolids and tillage practices influence soil bacterial communities in dryland wheat. Microbial Ecology. https://doi.org/10.1007/s00248-019-01339-1.
Schlatter, DC, Baugher, CM, Kahl, D, Huggins, DR, Johnson-Maynard, J, Paulitz, TC. 2019b. Bacterial communities of soil and earthworm casts of native Palouse Prairie remnants and no-till wheat cropping systems. Soil Biology and Biochemistry. https://doi.org/10.1016/j.soilbio.2019.107625.
Schlatter, DC, Reardon, CL, Maynard-Johnson, JL, Brooks, E, Kahl, KB, Norby, J, Huggins, DR, Paulitz, TC. 2019c. Mining the drilosphere: bacterial communities and denitrifier abundance in a no-till wheat cropping system. Frontiers in Microbiology. 10:1339.
Schlatter, DC, Kahl, K, Carlson, B, Huggins, DR, Paulitz, TC. 2020a. Soil acidification modifies soil depth-microbiome relationships in a no-till wheat cropping system Soil Biology and Biochemistry 149: 107939.
Schlatter, D, Yin, C, Hulbert, S, Paulitz, TC. 2020b. Core microbiome rhizospheres of dryland wheat are influenced by location and land use history. Appl Environ Microbiol 86:e02135-19.https://doi.org/10.1128/AEM .02135-19.
Schroeder, KL, Schlatter, DC, Paulitz, TC. 2018. Location-dependent impacts of liming and crop rotation on bacterial communities in acid soils of the Pacific Northwest. Applied Soil Ecology 130: 59-68.
Sessitsch, A, Brader, G, Pfaffenbichler, N, Gusenbauer, D, Mitter, B. 2018. The contribution of plant microbiota to economy growth. Microbial Biotechnology 11:801–805. https://doi.org/10.1111/1751-7915.13290.
Smith Becker JRP, Borneman, J, Becker JO. 2022. Hyalorbilia species associated with H. schachtii suppression in sugar beet production in the Imperial Valley of California. Manuscript in Preparation.
Stott, D.E. 2019. Recommended Soil Health Indicators and Associated Laboratory Procedures. Soil Health Technical Note No. 450-03. U.S. Department of Agriculture, Natural Resources Conservation Service.
Wang, X, Mavrodi, DV, Ke, L, Mavrodi, OV, Yang, M, Thomashow, LS, Zheng, N, Weller, DM, Zhang, J. 2015. Biocontrol and plant growth-promoting activity of rhizobacteria from Chinese fields with contaminated soils. Microbial Biotechnology 8:404–418. https://doi.org/doi:10.1111/1751-7915.12158.
Wang, L, Mazzola, M. 2019. Field evaluation of reduced rate Brassicaceae seed meal amendment and rootstock genotype on the microbiome and control of apple replant disease. Phytopathology 109:1378-139.
Washington State Department of Agriculture. 2023. Washington Soil Health Initiative. https://agr.wa.gov/departments/land-and-water/natural-resources/soil-health.
Weller, DM, Raaijmakers, JM, McSpadden Gardener, BB, Thomashow, LS. 2002. Microbial populations responsible for specific soil suppressiveness to plant pathogens. Annual Review of Phytopathology 40: 309-348.
Witte, H. Yang, J-I, Logan, GD, Colindres, NB, Peacock, BB, Smith Becker, J, Ruegger, PM, Becker, JO, Borneman, J. 2021. Hyalorbilia oviparasitica clade detected in field soils cropped to sugar beets and enriched in the presence of Heterodera schachtii and a host crop. PhytoFrontiers 1:13-20 https://doi.org/10.1094/PHYTOFR-07-20-0005-R.
Yang, JI, Benecke, S, Jeske, DR, Rocha, FS, Smith Becker, J, Timper, P, Becker, JO, Borneman, J. 2012. Population Dynamics of Dactylella oviparasitica and Heterodera schachtii: Toward a Decision Model for Sugar Beet Planting. Journal of Nematology 44(3):237-244.
Yang, M, Schlatter, DC, LeTourneau, MK, Wen, S, Mavrodi, DV, Mavrodi, OV, Thomashow, LS, Weller, DM, Paulitz, TC. 2022. Eight years in the soil: temporal dynamics of wheat-associated bacterial communities under dryland and irrigated conditions. Soil Biology and Biochemistry: submitted
Yin, C, Hulbert, SH, Schroeder, KL, Mavrodi, O, Mavrodi, D, Dhingra, A, Schillinger, WF, Paulitz, TC. 2013. Role of bacterial communities in the natural suppression of Rhizoctonia solani bare patch disease of wheat (Triticum aestivum L.) Applied and Environmental Microbiology. 79: 7428-7438.
Yin, C, Mueth, N, Hulbert, S, Schlatter, D, Paulitz, TC, Schroeder, K, Prescott, A, Dhingra, A. 2017. Bacterial communities on wheat grown under long-term conventional tillage and no-till in the Pacific Northwest of the United States. Phytobiomes. 1:83-90. https://doi.org/10.1094/PBIOMES-09-16-0008-R.
Yin, CT, Schlatter, DC, Kroese, DR, Paulitz, TC, Hagerty, CH. 2021. Responses of Soil Fungal Communities to Lime Application in Wheat Fields in the Pacific Northwest. Frontiers in Microbiology 10.3389/fmicb.2021.576763.
Zeng, Y, Stewart, J, Abdo, Z, Charkowski, A, Frost, K. 2019. Response of the soil microbiome to 1,3-dichloropropene application for nematode management. Phytobiomes https://doi.org/10.1094/PBIOMES-11-18-0055-R.