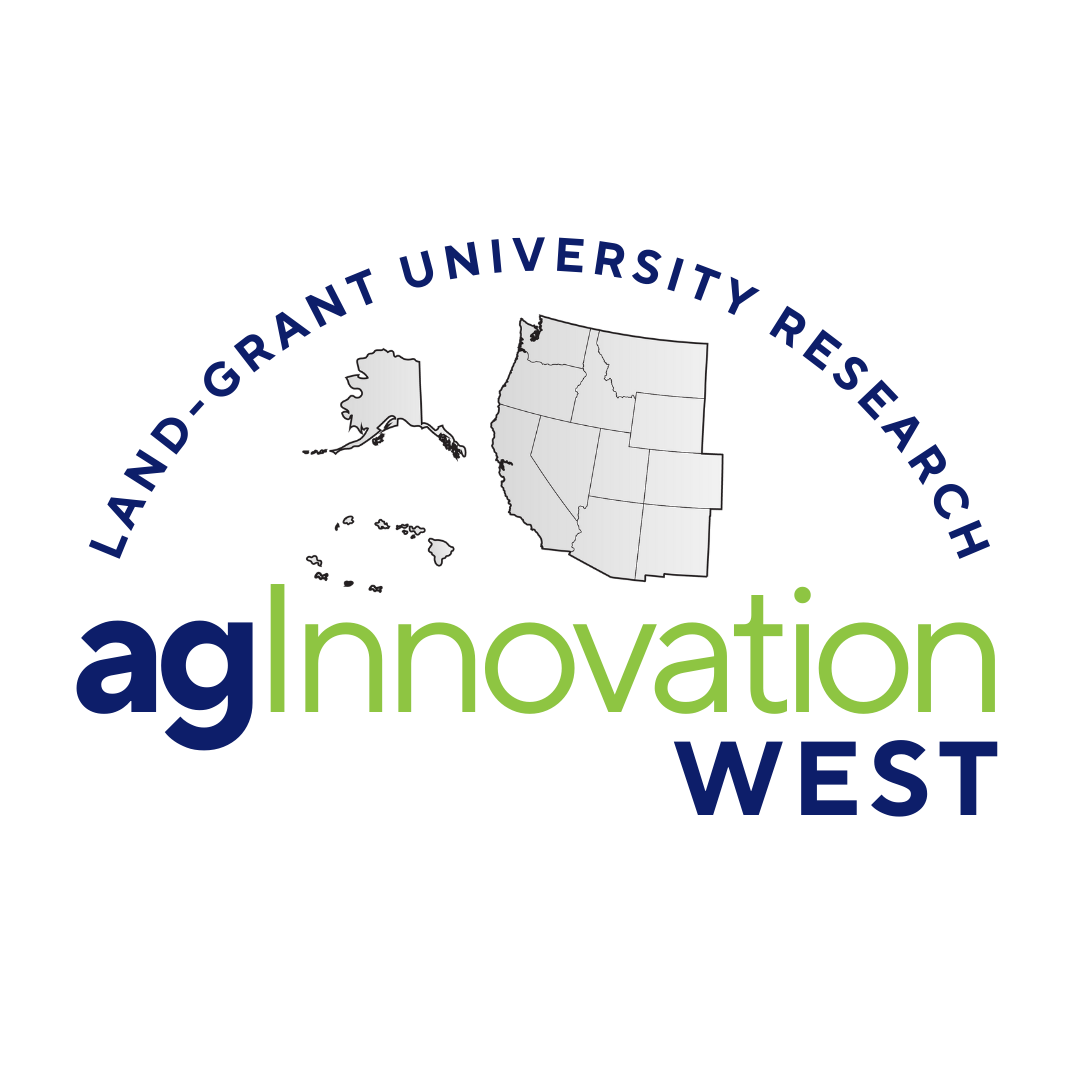
W4188: Soil, Water, and Environmental Physics to Sustain Agriculture and Natural Resources
(Multistate Research Project)
Status: Inactive/Terminating
W4188: Soil, Water, and Environmental Physics to Sustain Agriculture and Natural Resources
Duration: 10/01/2019 to 09/30/2024
Administrative Advisor(s):
NIFA Reps:
Non-Technical Summary
Statement of Issues and Justification
Soil is one of the most crucial components in supporting life, food security, and ecosystem services on Earth. As a key part of critical zone that determines agricultural and environmental sustainability, soils transform and supply water, energy, nutrients, and organic materials and moderate supply of water and nutrients for plants. Soil is where biological and chemical transformations occur, and it is the domain that sustains all flora and fauna ecosystem cycles. Meanwhile, changing societal food and energy demands, land use and climatic conditions are imposing ever greater stresses on soil. Recognizing the importance of soils for food security, agriculture, and in mitigation of climate change and sustainable development, the United Nations declared the International Year of soils, 2015 (IYS 2015) to raise awareness worldwide. The protection and stewardship of this crucial resource can be only assured through a better understanding of soil processes at different space and time scales.
Soil physics plays a critical role in understanding soil resources and functions, and it has made outstanding progress in recent years and not considering soil anymore as a homogeneous porous medium composed of various primary and secondary particle sizes but better understanding the roles of soil structure and flux heterogeneity across scales for compensating extreme events and anthropogenic impacts. Storage, redistribution, transport and transformation processes of water, heat and chemicals are understood for relatively small-scale systems in the vadose zone. However, Nielsen (1997) stated with regard to the vadose zone, “it is there but nobody cares.” Since then, much has been learned and documented in the “Vadose Zone Journal” among other esteemed outlets.
Whereas traditionally soil physics has largely been developed for and continues to contribute to agricultural sciences such as in irrigation management, nutrient management, and crop growth, its scope has evolved over the last 30 years, with relevant applications to many other disciplines in earth and environmental sciences, including ecology, hydrology, geology, biogeochemistry, engineering, climate science, and plant sciences. This trend of an evolving soil discipline is especially recognizable when reviewing this Regional Project over its past, as it has adapted from a strictly soil physics domain with fundamental physical applications towards transdisciplinary approaches and outcomes. This change has been critical as soil continues to undergo profound changes due to human activities such as land use change and agricultural intensification.
Despite considerable scientific advances in soil physics research in general and the efforts made by this multistate team in particular (described above), knowledge gaps still remain in measurement and modeling, transfer across spatio-temporal scales, and multidisciplinary integration of results. Without addressing these knowledge gaps, we would not be able to meet contemporary and emerging societal challenges and needs, and to respond and engage across the earth and environmental sciences in central issues such as climatic change, ecosystem services, food security, and energy production (see Wendroth et al., 2018 and contributions therein). To advance soil physics as a central solution for dealing with this multitude of socio-environmental challenges, we will develop and improve measurement tools and process-based models for quantifying soil ecosystem processes. Statistical analyses and modeling will help us to disentangle processes and their relationships at different scales to improve our ability to transfer information between scales. Finally, we will use our skills as soil physicists and environmental physicists and the knowledge we have gained through decades of studying this critical zone to advise and participate in national and international interdisciplinary projects to impart the importance and management of soil resources.
The collaborations created and fostered through the multistate research program have spanned generations of soil physicists and hydrologists, and it is the collective opinion of the participants that multi-institutional and multi-PI collaborations have been significantly enhanced because of the multistate program. Indeed, maintaining the focus of such a large group would not be possible without this multistate program. Using these collaborations, significant benefits have been realized through understanding soil physics principles and applying them to agricultural and environmental sustainability of soil resources, protecting ground and surface waters, improving agricultural production, and promoting ecosystem biodiversity, to name only a few areas. Members of this group tend to form and re-form around new multi-investigator programs to address emerging critical questions for sustainable solutions to grand challenges. This flexible and synergistic approach has been extremely productive, and it encourages a rich pollination of ideas and solutions to complex problems. The multistate committee structure is a convenient and efficient platform for establishing national research collaborations, validating approaches and techniques, pooling data, creating rigorous peer reviews, sharing equipment and developing the next generation of highly-trained soil scientists, environmental scientists, and engineers. This proposal seeks to maintain the ties between this extremely productive and creative group that, without the W4188 committee, would not be as focused on national needs research. The proposal also highlights our efforts to improve environmental monitoring, implement basic soil physics research, reach out to a broader scientific community (e.g., plant science, ecology, chemistry, and microbiology), and educate and communicate to stakeholders and colleagues within and outside our traditional disciplines.
Soil is one of the most crucial components in supporting life, food security, and ecosystem services on Earth. As a key part of critical zone that determines agricultural and environmental sustainability, soils transform and supply water, energy, nutrients, and organic materials and moderate supply of water and nutrients for plants. Soil is where biological and chemical transformations occur, and it is the domain that sustains all flora and fauna ecosystem cycles. Meanwhile, changing societal food and energy demands, land use and climatic conditions are imposing ever greater stresses on soil. Recognizing the importance of soils for food security, agriculture, and in mitigation of climate change and sustainable development, the United Nations declared the International Year of soils, 2015 (IYS 2015) to raise awareness worldwide. The protection and stewardship of this crucial resource can be only assured through a better understanding of soil processes at different space and time scales.
Soil physics plays a critical role in understanding soil resources and functions, and it has made outstanding progress in recent years and not considering soil anymore as a homogeneous porous medium composed of various primary and secondary particle sizes but better understanding the roles of soil structure and flux heterogeneity across scales for compensating extreme events and anthropogenic impacts. Storage, redistribution, transport and transformation processes of water, heat and chemicals are understood for relatively small-scale systems in the vadose zone. However, Nielsen (1997) stated with regard to the vadose zone, “it is there but nobody cares.” Since then, much has been learned and documented in the “Vadose Zone Journal” among other esteemed outlets.
Whereas traditionally soil physics has largely been developed for and continues to contribute to agricultural sciences such as in irrigation management, nutrient management, and crop growth, its scope has evolved over the last 30 years, with relevant applications to many other disciplines in earth and environmental sciences, including ecology, hydrology, geology, biogeochemistry, engineering, climate science, and plant sciences. This trend of an evolving soil discipline is especially recognizable when reviewing this Regional Project over its past, as it has adapted from a strictly soil physics domain with fundamental physical applications towards transdisciplinary approaches and outcomes. This change has been critical as soil continues to undergo profound changes due to human activities such as land use change and agricultural intensification.
Despite considerable scientific advances in soil physics research in general and the efforts made by this multistate team in particular (described above), knowledge gaps still remain in measurement and modeling, transfer across spatio-temporal scales, and multidisciplinary integration of results. Without addressing these knowledge gaps, we would not be able to meet contemporary and emerging societal challenges and needs, and to respond and engage across the earth and environmental sciences in central issues such as climatic change, ecosystem services, food security, and energy production (see Wendroth et al., 2018 and contributions therein). To advance soil physics as a central solution for dealing with this multitude of socio-environmental challenges, we will develop and improve measurement tools and process-based models for quantifying soil ecosystem processes. Statistical analyses and modeling will help us to disentangle processes and their relationships at different scales to improve our ability to transfer information between scales. Finally, we will use our skills as soil physicists and environmental physicists and the knowledge we have gained through decades of studying this critical zone to advise and participate in national and international interdisciplinary projects to impart the importance and management of soil resources.
The collaborations created and fostered through the multistate research program have spanned generations of soil physicists and hydrologists, and it is the collective opinion of the participants that multi-institutional and multi-PI collaborations have been significantly enhanced because of the multistate program. Indeed, maintaining the focus of such a large group would not be possible without this multistate program. Using these collaborations, significant benefits have been realized through understanding soil physics principles and applying them to agricultural and environmental sustainability of soil resources, protecting ground and surface waters, improving agricultural production, and promoting ecosystem biodiversity, to name only a few areas. Members of this group tend to form and re-form around new multi-investigator programs to address emerging critical questions for sustainable solutions to grand challenges. This flexible and synergistic approach has been extremely productive, and it encourages a rich pollination of ideas and solutions to complex problems. The multistate committee structure is a convenient and efficient platform for establishing national research collaborations, validating approaches and techniques, pooling data, creating rigorous peer reviews, sharing equipment and developing the next generation of highly-trained soil scientists, environmental scientists, and engineers. This proposal seeks to maintain the ties between this extremely productive and creative group that, without the W4188 committee, would not be as focused on national needs research. The proposal also highlights our efforts to improve environmental monitoring, implement basic soil physics research, reach out to a broader scientific community (e.g., plant science, ecology, chemistry, and microbiology), and educate and communicate to stakeholders and colleagues within and outside our traditional disciplines.
Related, Current and Previous Work
Though other active multistate research projects examine related soil, water quality, and water quantity issues, none of them focus on the interactions and feedbacks between soil physical and hydraulic properties, soil structure, energy and mass balances, soil health, and climate. Relevant multistate projects with some aspects similar to the proposed W4188 activities include:
· W3128: Scaling Microirrigation Technologies to Address the Global Water Challenge
· WERA103: Nutrient Management and Water Quality
- W3045: Agrochemical Impacts On Human And Environmental Health: Mechanisms And Mitigation
- W3170: Beneficial Reuse of Residuals and Reclaimed Water: Impact on Soil Ecosystem and Human Health
- W3190: Management and Policy Challenges in a Water-Scarce World
- W4147: Managing Plant Microbe Interactions in Soil to Promote Sustainable Agriculture
- NCERA217: Drainage design and management practices to improve water quality
- NE1438: Hydropedology of Vernal Pool Systems
- S1063: Quantification of best management practice effectiveness for water quality protection at the watershed scale
- NC1178: Impacts of Crop Residue Removal for Biofuel on Soils
- NC1179: Food, Feed, Fuel, and Fiber: Security Under a Changing Climate
The results of the previous W3188 multistate project are extensive, timely and applicable to numerous agricultural and environmental issues. With the national dialog further expanding to include impacts of climate change, links between population growth in the US and land use change, the need for sustaining the health of soils, and the importance of soil to moderate and control the water budget and important ecological systems, the general themes of W4188 are even more critical. There is consensus that the soil physics community can and should continue to pursue collaborative efforts, so that our thus-far integrated knowledge and skills can be applied to sustainable agricultural and environmental practices, natural resource stewardship, and the adaptation to and mitigation of global climate change.
The objectives of this multistate project are to: 1) Connect new understandings of storage and transport of mass and energy to assess environmental change; 2) Develop and test new methods and models to improve the quality of soil information and knowledge; and 3) Integrate scale-appropriate methods to improve decisions of soil and water resources. Concepts and previous work related to the objectives of this proposal are presented in the following text.
- Connect new understandings of storage and transport of mass and energy to assess environmental change.
1.1. Non-destructive imaging for understanding sub-visible scale processes
Non-destructive imaging methods such as X-ray Computed Tomography (CT) and neutron radiography (NR) yield high-resolution (2 microns), 3-D representations of soil pore space and fluid distribution that can be used in conjunction with advanced simulation techniques such as smoothed particle hydrodynamics (SPH) or the lattice Boltzmann (LB) model to significantly advance our knowledge about fluid distribution, interfacial phenomena, and flow and transport processes in soils. Ongoing and future research areas include improving scanning procedures and developing image segmentation and pore-space analysis tools for quantitatively characterizing soil pore space. A number of CT and NR systems are used today by multi-state researchers, ranging from benchtop scanners to synchrotron microtomographs. They primarily differ in X-ray source and energy, detector geometry, and sample manipulation capabilities. Comprehensive reviews about fundamentals of computed tomography are provided in Stock (1999), Ketcham and Carlson (2001), and Wildenschild et al. (2002). Some applications of CT and NR in porous media research include pore space characterization with respect to variables such as bulk density (Rogasik et al., 1999), volumetric water content (Hopmans et al., 1992; Rogasik et al., 1999), phase distributions (Wildenschild et al., 2002), breakthrough of solutes in porous media (Clausnitzer and Hopmans, 2000; Perret et al., 2000), pore-scale configuration of immiscible organic fluids in multiphase systems (Schnaar and Brusseau, 2005, 2006a,b), and rhizosphere water retention, distribution and flow (Carminati et al., 2010, Carminati, 2013, Zheng et al., 2018).
1.2. Characterization and description of physical properties of the vadose zone
Soil hydraulic properties are key to quantitatively describing soil water flow and chemical transport. Hydraulic properties of natural soils are scale dependent, time dependent, and spatially variable. In agricultural soils, temporal changes in soil hydraulic properties are primarily caused by tillage (Or et al., 2000), clay content and clay mineralogy, and water and soil quality. Changes in soil volume and pore space induced by clay swell-shrink processes present a challenge to developing predictive models for flow and transport, in particular to develop constitutive hydraulic functions. Such functions are important not only for design of man-made hydraulic barriers such as clay liners constructed for waste isolation, but also for fluid flow predictions in porous media (Mitchell, 1993; Benson et al., 1994). Advances in pore-scale modeling of fluid flow and liquid distribution in rigid angular pores have been developed by participants of this multistate program. These consider both capillarity and adsorption (Tuller et al., 1999; Or and Tuller, 1999; Masad et al., 2000; Tuller and Or, 2001; Tashman et al., 2003) and provide the basis for a proposed multiscale-modeling framework in soils. This framework has been extended to consider the effect of surface roughness and change in fluid properties (Zheng et al., 2015, 2018). Other methods for deriving hydraulic properties include pore-scale network models (Vogel, 2000; Vogel et al., 2005; Li et al., 2005), percolation-based methods (Hunt and Ewing, 2009), and lattice-Boltzmann methods (Vogel et al., 2005; Zhang et al., 2005; Schaap et al., 2007).
Simultaneous scaling of soil water retention and hydraulic conductivity functions provides an effective means to characterize the heterogeneity and spatial variability of soil hydraulic properties in a given study area. The statistical significance of this approach largely depends on the number of soil samples collected. Unfortunately, direct measurement of the soil hydraulic functions is tedious, expensive and time-consuming. CA is developing simple and cost-effective hybrid scaling approaches (Nasta et al., 2013) that combine the use of ancillary information (e.g. particle-size distribution and soil bulk density) with direct measurements of saturated soil water content and saturated hydraulic conductivity. Our results demonstrate that the presented approach requires far fewer laboratory measurements than conventional scaling methods to adequately capture the spatial variability of the soil hydraulic properties.
Thermal properties of porous media are important in many environmental and industrial applications. For instance, the thermal conductivity and heat capacity of soils greatly affect the partitioning of solar radiation into components of soil heating, the transfer of long-wave radiation, and sensible and latent heat transfer. The method of DeVries (1963) is commonly used for predicting the thermal properties from texture and phase content of porous media. More recent methods have used pore-scale modeling methods to relate the core-scale thermal conductivity to phase distributions (Hu et al., 2001; Ewing and Horton, 2007; Lu et al, 2014).
1.3. Multi-scale flow and transport including impacts from climate change
The design of spatial and temporal sampling schemes is based on several questions. How do we sense variation of a soil physical and related state variable or property? How do we separate measurement noise from signal? What are ways of transferring scale-specific soil physical information to different domains while maintaining important variance characteristics? Do the spatial or temporal covariance behavior manifest that our measurement design was adequate to solve the problem? Typically, measurements taken at the instrument scale are used for spatial or temporal processes at some larger domain (Ellsworth and Boast, 1996). For this purpose, a “good average” of the derived property is often applied with a “local-scale” model to make a large-scale prediction (Cahill et al., 1999). Averages can be misleading (Stockton and Warrick, 1971). For example, when averages fail to represent the horizontal variability structure (Nielsen et al., 1973), predictions over time may be fallacious when the local status deviates from the mean. Nielsen (1987) questioned, how can we integrate information from the measurement scale to our goal scales?
Investigations of the spatial and temporal variability structure of relevant soil physical state variables (soil water content) and related soil hydraulic functional properties (Western et al., 2004; Comegna and Vitale, 1993; Ünlü et al., 1989; 1990; Shouse et al., 1995; Adhikari et al.,2012; Stewart et al. 2016) reveal substantial changes of spatial correlation lengths of soil-water-related state variables with time and the magnitude of soil-water content (Roth, 1995; Wendroth et al., 1999; Vereecken et al., 2007, Green et al., 2007, 2009). Accordingly, the cross-correlation structure between soil water and related variables depends on soil water status (Nielsen et al., 1973; Greminger et al., 1985). Moreover, seasonal evolution of soil moisture variance and correlation length reoccurs during times of recurring water status (Western et al., 2004).
These more recent investigations cause us to revisit the original question posed by Nielsen (1987): Why is understanding spatio-temporal variability of soil moisture and related transport and transformation coefficients so important? This question can be applied to many scale-dependent processes, including estimating soil water processes in space and time, evaluating bioavailability of soil contaminants, classifying functional and eco-physical soil and landscapes, establishing scale hierarchy of soil physical properties and regionalization, and scale transferring soil water processes. Nielsen’s question and these applications highlight the need to estimate regional distributions of soil water status and related transport coefficients, fluxes, and transformation coefficients at different size domains with sufficiently fine resolution to capture management and geochemical impact on variable magnitude (Robinson et al., 2008a).
1.4. Applications of soil physics and biophysics in ecology and agriculture
Although soil physics and biophysics have addressed soil-plant-atmosphere continuum (SPAC) issues for many decades (Russell, 1960; Campbell, 1977; Wraith and Baker, 1991; Campbell and Norman, 1998; Kirkham, 2005), the root zone, as one of the most critical components of the SPAC, is the least understood (Lazarovitch et al., 2018). Whereas conceptual and numerical models that incorporate root water uptake and root response have been developed (Simunek and Hopmans, 2009, Hartmann et al., 2017, Cai et al., 2017), experimental studies are needed to test the hypotheses regarding mechanisms of various processes involved. The main message from the 2016 Kirkham Conference was that more experimental and modeling efforts are needed to better understand and quantify the complex interactions operative at multiple scales within the soil root zone and to do so while building bridges to other research communities and disciplines (Lazarovitch et al., 2018). This multistate group is uniquely qualified to meet these challenges. Two decades ago, the term ecohydrology was coined and predictions of major breakthroughs and intensive activity in understanding spatio-temporal soil-plant-climate interactions were made (Zalewski et al., 1997; Baird and Wilby, 1999; Rodriguez-Iturbe, 2000). Today ecohydrology is a thriving discipline with soil physics as a major constituent informing research across scales from soil-nutrient-root interactions to desertification (Wardle et al., 2004; Hopmans, 2006; Reynolds et al., 2007). Recent advances have been made applying principles of soil physics to vegetation and soil patterns (Robinson et al., 2008b), desert ecosystems (Shafer et al., 2007; Wang et al., 2007; Adhikari et al.,2012), Pinion-Juniper woodlands (Lebron et al., 2007; Shukla et al., 2006), soil microbial habitat (Or et al., 2007), soil biophysics (Smucker and Hopmans, 2007), urban vegetative function (de la Mota et al., 2018), and other topics.
Soil water cycle in the rhizosphere, where large communities of microbes reside, is impacted by pore-scale interactions of water with extracellular polymeric substances (EPS) and biofilms, the 3D structures built with EPS as the scaffold. These interactions cause time dependent changes in interfacial energy, viscosity and solute connectivity (Roberson & Firestone, 1992; Volk et al., 2016; Zheng et al, 2018)) and further impacts on root and microbial access to nutrient (Rosenzweig, 2013), evaporation processes (Deng et al., 2015; Zheng et al., 2018), colloid transport, and during stages of biofilm dehydration impacts interfacial energies leading to seasonal water repellency (Schaumann et al., 2007; Achtenhagen et al., 2015) and poor infiltration (Aminzadeh and DiCarlo, 2010). Recent experiments demonstrate that biofilm changes evaporation rates, decreases hydraulic conductivity, and decreases gravitational drainage, but also benefit soil health and plant productivity by increasing plant available water and increasing the energy potential at which that water is available (Zheng et al., 2018).
Soil water repellency is a common occurrence after wildfires, which can affect watershed hydrology (DeBano, 2000; Parise and Cannon, 2012; Moody et al., 2013) and can lead to significant ecological and socio-economic impacts (Hallema et al., 2018). Links between post-fire soil water repellency and hydrological processes such as infiltration, runoff and erosion are frequently measured (Hubbert et al., 2006; Moody et al., 2009; Ebel and Moody, 2016) but poorly understood. For example, we are just beginning to understand how water repellency is related to infiltration through sorptivity. Shillito et al. (2019) showed how increased water repellency will functionally decrease sorptivity and, ultimately, infiltration.
1.5. Transport and transformations of solutes, nanoparticles, and emerging contaminants
Emerging contaminants, such as hormones, pharmaceuticals (including antibiotics), antibiotic resistant bacteria (ARB) and antibiotic resistance genes (ARGs), have been frequently detected in surface water, groundwater, soils, sediments, and agricultural produce worldwide (Kolpin et al., 2002; Qin et al., 2015, Williams-Nguyen et al., 2016). Their ubiquitous presence in agroecosystems results from the wide use of pharmaceuticals in human healthcare and animal agriculture, and subsequent environmental contamination via wastewater discharge, land application of animal manure and sewage sludge, and crop irrigation with reclaimed waters (Kumar et al., 2005; Lee et al., 2007; Malchi et al., 2014). A wide variety of antibiotics are found in perhaps 50% of US surface waters with concentrations up to 1 µg/L (Kolpin et al., 2002). The levels and prevalence of ARB and ARGs are also rising rapidly (Spellberg et al., 2016), which can adversely impact human and ecosystem health, and agricultural productivity (Williams-Nguyen et al., 2016). In particular, food crops grown with contaminated soil and water could become hot spots of the exchange and fluxes of antibiotics, ARB, and ARGs, which may affect the development and proliferation of ARB and ARGs. Pathogens, which include viruses, bacteria and other microorganisms, are also creating major challenges as water resources dwindle and wastewater is reused. These emerging contaminants, pathogens and metabolites present unique challenges in understanding their fate and transport in the soil-water environment. Many emerging contaminants are potent at very low concentrations and labile, particularly during preferential flow events (Radolinski et al., 2018), yet can also associate strongly with soil solids and undergo rapid and complex transformations. Specialized laboratory experiments together with modeling and field observations are required to fully understand their fate and transport in the vadose zone (Fan et al., 2007a, b). Often these compounds (pesticides, radionuclides, and metals) can strongly associate with soil colloid particles (≤ 10μm in size), which can significantly reduce mobility and increase persistence (McGechan and Lewis, 2002; Bradford et al., 2003; Bradford and Torkzaban, 2008; Xing et al., 2016; Li et al., 2019). Soil pores might have a large control on the interactions between nanosized viruses and microsized bacteria in structured soils under redox and drying-wetting cycles, thus influencing soil microbial community and associated carbon and nitrogen transformation (Liang et al., 2019). Understanding colloidal processes in soils and sediments is important for environmental quality and soil health. We need to understand the mechanisms of colloid retention at different interfaces and pore systems to make accurate predictions of colloid transport, colloid-facilitated transport of contaminants, nutrients, and other colloid associated entities such as soil carbon (Xu et al., 2019). Many industrial and consumer products are increasingly using ENPs, such as textile, paint, pesticides, sunscreen, cosmetics, medicine, and food additives (Kah et al., 2013; Kah and Hofmann, 2014; NRC, 2012). In particular, the release of ENPs from nanopesticides use may be much greater than the combined release from all other sources (e.g., 54 times greater for nano-Ag and 2,232 times greater for nano-TiO2) (Gogos et al., 2012). Thus, environmental concentrations of ENPs will increase rapidly (Gottschalk et al., 2009, 2010). Then crop plants are exposed to ENPs due to sewage sludge application in agricultural land and the use of nanopesticides for crop protection (Gardea-Torresdey et al., 2014; Larue et al., 2014), which may result in uptake and accumulation of ENPs in plant (Gardea-Torresdey et al., 2014; Ma et al., 2010; Rico et al., 2011). Therefore, consumption of food crops (including fresh produce) becomes an important human exposure route to ENPs (Ma et al., 2010; Miralles et al., 2012; Rico et al., 2011). Chronic human exposure to trace-level ENPs through dietary intake warrants precaution and future research (Gardea-Torresdey et al., 2014; Miralles et al., 2012). Emerging contaminants, such as ENPs, pharmaceuticals, ARB, and ARGs, are not commonly monitored or regulated, and may negatively impact human and ecosystem health (Sauvé and Desrosiers, 2014).
One group of persistent organic contaminants (per- and polyfluoroalkyl substances [PFAS]) has grabbed national spotlight due to the contamination of groundwater, surface water and drinking water as well as their known ubiquity, persistence, bioaccumulation and toxicity to humans. PFAS have been widely used in many products such as carpets, clothing, furniture fabrics, food packaging, and water-, grease- or stains-resistant cookware (Guelfo and Adamson, 2018). PFAS are also used in firefighting foams and numerous industrial and commercial applications. PFAS such as perfluorooctanoic acid (PFOA) and perfluorooctanesulfonic acid (PFOS) are extremely stable and are therefore the most common PFAS found in the environment. The concentrations of PFOS and PFOA in drinking water supplies for 6 million U.S. residents exceeded the limit of US EPA’s lifetime health advisory level (70 ng/L) reported in a recent survey between 2013 and 2015 (Hu et al., 2016; Hurley et al., 2016). PFAS containing longer alkyl chains tend to be more bioaccumulative, and possibly toxic to humans and wildlife. Animal studies report the toxicity of PFAS to the liver, immune and endocrine systems, as well as the developing fetus and neonate. As PFAS may remain in wastewater effluents and sewage sludge (biosolids) (Appleman et al., 2014; Bossi et al., 2008), the common agricultural practices of crop irrigation with wastewater effluents and land application of biosolids for fertilizer values undoubtedly introduce many PFAS into agroecosystems and potential bioaccumulation of PFAS in agricultural products.
The transport, retention, and degradation of organic chemicals (e.g., antibiotics, pesticides, and plastics) determine their fate in soils and impacts on soil health. However the complicated relations of transport, retention and degradation have not been elucidated from an integrated perspective as to the roles of soil surface, pore system, and water dynamics. Small angle scattering (SAS) techniques have been widely applied to study pore structure in a variety of materials, such as petrographic samples (Radlinski et al., 2004; Sastry et al., 2000), wood charcoals (Venkatraman, 1996), porous alumina (Rasmussen, 2001), and clay minerals (Bihannic et al., 2001). Compared with other techniques, SAS enables the ability to study a wide range of void sizes (from ~10Å to 15-μm), and provide non-intrusive information on the entire porosity, not just that portion that is accessible from the exterior. Over length scales of 10- to 5,000-nm, ultra-small angle X-ray scattering (USAXS) can provide new information about total porosity (including the interior and blocked portions), pore size distribution, and the size distribution of SOM-filled pores (McCarthy et al., 2008). Ultra-small angle neutron scattering (USANS) could provide analogous information on the size distribution of water- and organics-containing pores. The combined use of USAXS and USANS could thus be a powerful approach to examining the transport, retention, and degradation of organic contaminants as a function of soil pore size distribution, particularly with respect to the coupled impacts of SOM-mediated pore system and water dynamics.
- Develop and test new methods and models to improve the quality of soil information and knowledge.
2.1. Improved multifunction measurement devices
Throughout large segments of the terrestrial sciences, including agriculture, ecology, and hydrology, there is a pressing need to improve instruments to better interrogate subsurface environments, especially those capable of providing data for multiple state variables, which affect water movement, nutrient dynamics, plant root behavior, microbial community, and temperature profiles. Recent publications highlight the improvements and the broader importance of such technologies toward answering multi-disciplinary questions (Ferré and Kluitenburg, 2003; Jones and Shenai, 2007; Marchant and Arrouyas, 2014). These new multi-function probes will be field and laboratory tested. Nonetheless, many of these approaches are still under development and will be improved upon.
2.2. Quantifying near-surface processes with instruments and analyses
Net ecosystem production and net ecosystem exchange are closely tied to soil properties. Researchers have a significant opportunity to assist the agricultural and ecological communities by addressing the importance of soil properties and processes. Heitman et al. (2008) showed an approach that uses heat pulse probes as a means to estimate latent heat flux, a critical component of the energy budget. Upscaling these point-scale values to basin-scale (Zhu et al., 2006) would allow better assessments of regional-scale water status, yet advancement of instrumentation and analyses has lagged the potential societal applications. In particular, remote sensing tools are becoming more sophisticated, but ground truthing is needed.
2.3. Quantifying soil structure
Quantifying soil structure has been a challenge ever since soil structure has been recognized as an important part of soil physical properties (Baver, 1940, Kubiëna, 1938). In recent years, non-destructive imaging techniques such as X-ray computed tomography (CT) (Duliu, 1999, Taina, Heck, et al., 2008), nuclear magnetic resonance imaging (NMR) (Hemminga and Buurman, 1997) and neutron radiography (NR) (Oswald et al., 2008) have become available, and these allow the study of soil structure non-destructively and non-invasively. Despite this considerable progress form the imaging side, relating soil structure to physical properties and processes remains a challenge (Logsdon et al., 2013). As a consequence, soil structure information is still missing in most pedo-transfer functions (PTF) (Schaap et al., 1998; 2001). The temporary roles and impacts of non-stable soil structure under reduced tillage on soil transport process and rooting process remain unclear (Guan et al., 2015). Another deficiency is the lack of incorporating spatial covariance (Wendroth et al., 2006) although one of the main goals of PTFs is regionalization of soil hydraulic properties.
2.4. Application of machine learning methods
Within the era of Artificial Intelligence, Machine Learning provides a new generation tool to understand the world from a data perspective. Both classic and novel machine learning tools are created to help humankind unravel complicated natural systems as well as to advance the development and operation of products in industry.
For hydrologists and water resources scientists, a boost in the application of machine learning techniques has been initiated and it is reasonable to expect more increasing activity into the future (Marçais and de Dreuzy, 2017). The accessibility of large volumes of environmental data proliferates unbounded possibilities, while at the same time it becomes increasingly difficult to identify and interpret the valuable information that might be found in the data. Being able to find trends and other valuable relations within a set of environmental data is a main task of machine learning techniques.
As one of the most value natural resources, groundwater quality assessment is critical. Groundwater contamination throughout the Central Valley aquifer, California, was predicted by a hybrid machine learning model called boosted regression tree (Ransom et al., 2017). Support vector machine (SVM), and ANN (artificial neural networks) have also been applied for producing groundwater risk maps (Sajedi-Hosseini et al., 2018; Barzegar et al., 2018). For reservoir management, the surface water quality assessment is important as well. Trained with a long history data record, ANNs, SVM were applied for predicting Carlson’s Trophic State Index for reservoirs in Taiwan. These techniques manifest a significant improvement (Chou et al., 2018) in ability to interpret monitoring data.
As a newly emerging branch of machine learning, deep learning has gained popularity in both industries and the academic world during the last decade. To name a few, image classification and speech recognition are two fields where deep learning has been most successfully applied. The state of art performance by deep learning techniques is driving breakthroughs in those fields (Shen, 2017). Scientists have also put efforts to interpret deep learning by visualizing their framework (Zeiler and Fergus, 2014) and to push their application progress in various domains, such as computational chemistry (Goh et al., 2017), medical imaging (Greenspan et al., 2016), and hydrology (Tao et al., 2016).
Deep learning pushes the frontier in hydrology related fields. As one deep neural network, SDAE (stacked denoising autoencoder) has been applied for retrieving precipitation from remote sensing images by bias correction (Tao et al., 2016). CNN (convolutional neural network) has shown an outstanding ability to detect the extreme events by classifying thousands of climatology images (Liu et al., 2016). Field-measured soil moisture has also been predicted by DBN (Deep Belief Net) (Song et al., 2016).
As one prospective direction of deep learning for water science, deep learning is applied in model calibration. Innovations in the deep learning framework has already demonstrated preliminary success for this task. Physics-guided neural networks have accomplished an excellent achievement in lake temperature modelling calibration. It does not only match the lake temperature measurement data but is also in accordance with other physical principles (Karpatne et al., 2018).
- To integrate scale-appropriate methods to improve decisions related to the management of soil and water resources.
The scale-appropriateness of a methodology, whether measurement or model, is based on the methods ability to provide measurements or calculations (soil water content, matric potential, water flux, heat flux, etc.) that capture the continuity of a process in the spatial or temporal domain, or both. The continuity of a spatio-temporal process needs to be derived, not only for assuring spatial representativeness of observations, but also to transfer the process of one or several variables across scales.
Much has been done to develop methodologies that will upscale measurements made at point scale to the larger scale. A review of the state-of-the-art of such methods as applied to soil moisture monitoring is presented by Oschner et al. (2013). Numerous examples also exist for methods to upscale pore-scale characterization of porous media to the core scale (Or and Tuller, 1999), and from core scale to field scale (Yeh et al., 1985; Zhu et al., 2006).
Capabilities to model large scale systems have improved greatly over the past decades because of vast improvements in computer speed as well as vastly improved numerical algorithms. In 1970, R.A. Freeze (1970) reported the simulation of watershed hydrology using 30,000 grid cells. In a recent example Kollet et al. (2010) demonstrated the ability to efficiently model the subsurface flow driven with land surface processes in a hypothetical watershed discretized with 8 x 109 grid cells using up to 16,384 parallel processors. Rather than using models that treat processes at fine scale (Darcy scale for instance), as with the Freeze (1970) and the Kollet et al. (2010) applications, an alternative is to use upscaled equations that are appropriate for field or subwatershed scale. An application of this type is outlined by Reggiani and Shellekens (2003), who describe the Representative Elementary Watershed approach to modeling of mass and energy transport in watersheds.
Objectives
-
1. Connect new understandings of storage and transport of mass and energy to assess environmental change.
Comments: See attachment. -
2. Develop and test new instrumentation, methods and models to improve the mechanistic understanding of soil processes and the quality of soil information and knowledge.
Comments: See attachment. -
3. Integrate scale-appropriate methods to improve decisions related to the management of soil and water resources.
Methods
Objective 1. Connect new understandings of storage and transport of mass and energy to assess environmental change.
1.1. Non-destructive imaging for understanding sub-visible scale processes
CA will design a two-dimensional split-root study, allowing for partial soil root zone water, nitrate, and temperature stresses, while measuring plant activity through total water use (ET). Innovative techniques to measure soil water and nutrient status will be neutron tomography and application of innovative HPP (heat pulse probe) developed under Objective 2.
AZ, NV, and CA will continue ongoing collaborations on use of X-ray CT and neutron tomography. Our focus is on solving questions related to the basic understanding of how the soil fabric affects water flow and contaminant transport in subsurface environments. Multistate researchers will acquire and reconstruct X-ray CT image for spatial analysis.
WY, CO, ID and AZ will evaluate different electromagnetic techniques to measure soil variables such as water content, water potential, and bulk electrical conductivity in larger basins and watersheds.
TX will continue working on in situ, air-borne, and space-borne sensors for understanding soil hydrologic processes and related parameterization across various space and time scales under different hydro-climatic conditions (Ines et al., 2013; Mohanty et al., 2013; Mohanty, 2013; Shin et al., 2012).
1.2. Characterization and description of physical properties of the vadose zone
TX will develop a comprehensive coupled water and heat transport numerical model including multi-domain unsaturated zone water flow and heat transport processes at continuum scale. This will further improve our capacity to better understand the water and energy cycle as well as soil moisture retrieval algorithms for remote sensing platforms.
NCSU and IA will use a coupled heat and water transfer numerical model to study the effects of time dynamic bulk density changes on surface energy partitioning and on subsurface temperature and water content. The numerical model will employ thermal and hydraulic properties as a function of bulk density and water content (Lu et al., 2016; Tong et al., 2016; Liu et al., 2017; Tian, et al., 2018a; Sadeghi et al., 2018; He et al., 2018; Kojima et al., 2018; Lu et al., 2018; Tian et al., 2018b).
NM will work on determination of the thermal properties of different soils irrigated with low salinity irrigation water and saline and sodic treated wastewater. Thermal properties will also be determined for soils irrigated with highly saline groundwater and concentrates. The application of saline water and the attendant changes of soil thermal properties will be studied, their effect on the survival and growth of horticulture and desert plants (Flores et al., 2016) and pore clogging, using image processing, will be quantified. Using numerical models leaching of nitrates and effects on root water uptake due to abiotic stresses will be quantified. This work will be done in collaboration with CA.
UT, AZ, and CA will study relationships between soil and manure physical properties and greenhouse gas emissions and evaporation. New inverse estimation schemes with uncertainty will be developed in collaboration with, CA and AZ. These researchers will estimate effective soil hydraulic and thermal properties across the USA at different resolutions by assimilating soil moisture from ground, air, and space-borne sensors.
IA, NV, KS and ND will analyze shallow soil temperature gradients and thermal properties in order to estimate soil water evaporation across scales as a function of depth and time.
VA will characterize the structural and hydraulic properties of soil through the use of methods including stable isotope analysis.
WA will study the effects of plastics on soil health, and in particular, the effects of plastics on soil physical properties. WA will develop methods to extract, quantify, and characterize plastics in soils, with an emphasis on nano- and microplastics.
KS will study the use of soil color measurements to capture the soil spatial variability of agricultural fields. A new highly portable soil color sensor for rapid field and laboratory observations can offer a first order approximation for identifying major soil types and describing soil profiles objectively.
1.3. Multi-scale flow and transport including impacts from climate change
CA and VA will collaborate with local investigators, measuring core-scale soil hydraulic properties, and apply scaling techniques to the hillslope scale. Measurements of soil water tension and soil moisture, in concert with piezometer data will be coupled with HYDRUS to simulate hillslope-scale soil hydrology.
VA will quantify temporal variability of soil hydraulic properties at the core and field-scale. This will combine field and laboratory measurements to understand small-scale processes with numerical and analytical models to characterize larger-scale responses. Emphasis will be placed on understanding the role of moisture-dependent preferential pathways in structured soils.
KY will study soil nitrogen dynamics in a farmer’s field under different crops with special emphasis on landscape position and nitrogen fertilizer supply. Soil water content, soil temperature, C and N gas emissions, soil mineral nitrogen concentration, nitrate concentration in leachate, crop nitrogen uptake and biomass yield will be measured. KY will study the usefulness of pedo-transfer functions at the field-scale and explore adaptations necessary.
ND will evaluate hydraulic responses of cracking, smectitic soils to freezing and thawing conditions in the Red River Valley of the North. ND will evaluate soil salinization on water budgets in agricultural clay soils.
1.4. Applications of soil physics and biophysics in ecology and agriculture
UT and ID will design and model plant growth systems for reduced gravity conditions based on porous medium physical properties and plant physiological characteristics.
ND will quantify soil porosity, shrinking and swelling, thermal properties, and water fluxes and their relationships to small-scale temporal shifts and cyclical patterns in microbial community structures and activity.
WY will quantify coupled water flow and heat transport in rangeland and forest soils and study the impact of disturbance on water, heat, carbon, and nitrogen fluxes.
CA continues working on understanding root zone soil hydrology and spatiotemporal variabilities in soil hydraulic properties using a variety of lab and field measurement techniques as well as computer modeling approaches. The soil moisture and soil matric potential data will be collected primarily from multiple landscape irrigation research trials. Undisturbed soil samples will be collected from all experimental sites and these data will be used to evaluate the performance of the existing pedotransfer function models and develop new machine learning based models [Haghverdi et al., 2018].
DE and OR are investigating the role of biofilms on the moisture retention curve. DE and OR will continue to quantify the physical properties of biofilms in soils and the impact these properties have on the moisture characteristic relationship. Laboratory results will be compared to field scale data to elucidate the relevance of these changes to ecosystem level processes.
NV, OR, DE, CA and MN will collaborate to better understand the relationships between soil water characteristics and interfacial energies of the soil solids as well as the process of infiltration under conditions of varying degrees of water repellency and moisture content.
WA will use soil physical tools to evaluate conditions that are conducive to microbial life in the driest places on Earth. Water contents and water activity will be measured in a variety of microenvironments in the Atacama desert, one of the driest places on Earth. Samples will be taken from the Atacama desert and then analyzed in the laboratory in WA. Water activity and water sorption isotherms will be determined and correlated to microbial signatures measured on the same samples.
KS will investigate the application of new biodegradable polymers to the soil surface with the goal of reducing evaporative losses while still allowing rainfall infiltration This study will make use of soil columns in laboratory and greenhouse to study changes between different soil types, biopolymer rates, and concentrations.
TN will focus on the effects of soil physical conditions on soil viral ecology. Specifically, the roles of soil water content, surface area, and soil structure in influencing viral transport, abundance, and biodiversity will be addressed in relation to bacterial abundance and biodiversity. Main experimental tools include isotope technique, rapid PCR, metagenomic analysis, and contrast match technique.
1.5. Transport and transformations of solutes, nanoparticles, and emerging contaminants
WA, CA, DE, VA, AZ, ND, TN, and MI will investigate interactions of nanoparticles with interfaces, and fate and transport of potential agricultural and emerging contaminants by conducting a combination of laboratory batch-type experiments, column experiments, numerical modeling, lysimeter experiments, greenhouse studies, and field-scale research. Nanoparticles in porous media interact with the solid-liquid and liquid-gas interfaces under varying flow and geochemical conditions. This group will use microscopic and macroscopic techniques to investigate and quantify the interactive processes. We will also use theoretical calculations to quantify interaction forces. Identifying and quantifying the mechanisms controlling nanoparticle fate and transport in the vadose zone will be incorporated into mathematical models (HYDRUS). ND, VA, MI, and CA will also use laboratory methods, primarily column breakthrough curves, batch sorption experiments, soil microcosm batch studies to examine the processes which control bioactive chemical fate and transport. Controlled experiments and observations from the field using soil extracts, lysimetry, and wells will be used to identify field fate and transport mechanisms.
CA, ND, DE, NV, TN, and MI will focus on characterizing the fate and transport of trace organic compounds (e.g., endocrine disrupting compounds) originating from reclaimed wastewaters, sewage sludge and animal wastes in soil and water through field, laboratory, and numerical experiments (batch and column scale to field scale). AK with USDA-ARS will conduct experiments on the attenuation of herbicides (2,4-D, triclopyr, and glyphosate) in sub-arctic soils incorporating lysimeters to distinguish between different attenuation modes. DE will conduct laboratory experiments and collecting wetland water and soil samples to elucidate the mechanisms of colloid release under dynamic redox conditions and determine the role that colloids play in nutrient transport and carbon cycling. VA will integrate laboratory and column experiments to quantify the role of preferential flow in subsurface transport of manure-derived antibiotics. MI will investigate fundamental interactions of pharmaceuticals and PFAS with soil and plant interfaces using batch sorption experiments, and explore their transport and uptake using column experiments and greenhouse growth experiments. MI will also use microbiological culturing, high throughput qPCR and sequencing techniques to characterize the composition and transport of ARB and ARG in soil, water and plant systems.
CO will develop a simulation model for methane fate and transport in soil. A critical task of this work will be characterizing the soil biology of methane producers and consumers, particularly in response to changing soil water content and temperature. This will be accomplished through laboratory batch methods and field flux chambers. Using controlled laboratory and field experiments, TX will focus on understanding the linked hydrologic and biogeochemical processes in the vadose zone (Hansen et al., 2011; Arora et al., 2013). Findings will be upscaled to plume scale and further implemented in coupled models such as HP1. TN will use SAXS and SANS techniques to investigate colloid-mediated movement and retention of emerging contaminants (e.g., pharmaceuticals and personal care products) at pore scales (nanoscale to microscale) under drying-wetting cycling conditions. In addition, IVIS bioluminescent imaging system will be used to quantify pathogen transport and profile distribution in heterogeneous soils under varying water saturation and steady/non-steady flow conditions.
Objective 2. Develop and test new methods and models to improve the quality of soil information and knowledge.
2.1. Improved multifunction measurement devices
IA and NCSU will develop a thermo-TDR pulse sensor to determine shallow soil profile temperature, thermal properties, soil water content, soil water flux, and sensible and latent soil heat fluxes.
TX will continue evaluation of the Cosmic Ray Soil Moisture Observing System (COSMOS) in a 5-ha subsurface drip irrigated field with cotton and corn as alternating crops in successive years. The COSMOS system will be compared with networks of neutron probe and wireless electromagnetic (EM) soil water sensors to understand the sensitivity of COSMOS to: 1) irrigation water supplied by subsurface drip irrigation at 0.30-m depth, 2) water supplied at the surface by irrigation, and 3) water contained in green biomass. In later years, COSMOS ability to linearly integrate over space will be tested by inducing patchy soil water variability by applying various levels of full and deficit irrigation in the ten management zones in the field. CA will continue to evaluate the potential of using small footprint cosmic ray probes in combination with in-situ neutron probes to estimate evapotranspiration of individual trees in almond and walnut orchards irrigated using micro-sprinklers or drip irrigation.
2.2. Quantifying near-surface processes with instruments and analyses
UT and AZ will develop multi-functional measurements for soil property determination including physically-based approaches for predicting evaporation rates from thermal surface signatures. IA and NCSU will measure heat and water fluxes in soils that experience time changing bulk density. They will work to develop a novel, physically-based approach for predicting liquid water fluxes and evaporation rates from thermal surface signatures. These rates will be tested (with NV) using thermal surface signatures and weighing lysimeters recently installed in NV and operated by DRI.
KY will continue the implementation of active optical crop sensors (GreenSeeker) to improve nitrogen fertilizer application schemes with special emphasis on landscape position. The results should inform to what degree nitrogen fertilizer application rates can be based on landscape topography. A capacitance probe will be used in this study for soil water content monitoring and its calibration will be refined (Wendroth et al., 2013).
NM will examine the soil moisture content using different sensors and soil temperature, and assess the usefulness of these sensors for measuring in situ soil salinity. Numerical models will be utilized to model soil water and solute dynamics through the vadose zone (Deb et al., 2013; 2015).
CA and many of the other participants in this multistate program (USSL, UCD, WSU, UDel, NDSU and DRI) will continue developing numerical tools to study and evaluate environmental processes and biogeochemical reactions across scales. This research will use HYDRUS to describe processes at multiple scales, including colloid and colloid-facilitated transport; preferential flow and transport of various chemicals (viruses, colloids, and bacteria); coupled biogeochemical reactions; coupled movement of water, vapor and energy; and micro-irrigation management practices on soil leaching.
MT will implement techniques to separate soil evaporation from plant transpiration directly from eddy covariance measurements at the plot scale in collaboration with scientists from the USDA. Bare soil and different cropping sequences will be studied including flood and pivot-irrigated crops to measure irrigation efficiency and to gain insight into the depths at which moisture is removed from soil by roots.
2.3. Quantifying soil structure
NV, AZ, KY, VA and ND will examine soils of different texture with respect to soil structural indices to improve the indirect estimation of soil functional properties (hydraulic conductivity function). ND will also examine soils of different sodium absorption ratios and ionic strengths. This task is relevant for improving PTFs. Here, auxiliary variables in combination with PTFs and their spatial covariance structure will be investigated with respect to their support of capturing field scale spatial variability of soil hydraulic properties (Reyes et al. 2018).
2.4. Application of machine learning methods
MN, CA, KY, AZ, TX and NV will apply Machine learning (ML) methods to quantify patterns in acquired data and to improve calibration of physically-based models. Example applications include the development of improved pedo-transfer functions, analysis of massive data bases for nitrate concentrations in aquifers and streams, and improved calibration of the SWAT model and the SMART model for watershed hydrology and water quality simulation.
Objective 3. To integrate scale-appropriate methods to improve decisions of soil and water resources.
We will continue developing interdisciplinary research programs and meetings (e.g., International Soil Modeling Consortium) and review publications (Vereecken et al., 2016). Experimental methodologies and instruments (large-scale soil property determination) and sampling designs targeted at geostatistics (Isaaks and Srivastava, 1989) and applied statistical time series analyses (Shumway and Stoffer, 2000) will be adapted and developed for agricultural and ecological applications (Nielsen and Wendroth, 2003).
CA will further evolve computational methodologies to characterize high-resolution basin, continental, and global scale transfer processes of salts and nutrients between the cultural landscape and groundwater through the vadose zone, to improve our understanding of the role of soils vis-à-vis anthropogenic activities and the impact to long-term dynamics of groundwater quality and groundwater-dependent surface water systems.
Across a network of micro-irrigated agricultural fields, with most to them in tree and other crops, CA and NM will deploy state-of-the-art subsurface monitoring capabilities for soil moisture, soil nitrate, salinity, and leaching of water and nitrate. The main objective of this field-scale research is to develop improved irrigation management practices that minimize nitrate losses while maintaining crop production. Field measurements will be coupled with numerical modeling tools (HYDRUS) for the main purpose to evaluate best management practices. The CA PIs are working with Cooperative Extension Specialists and Farm Advisors to conduct a large number of irrigation workshops to conserve water and sustain irrigated agricultural production. CA and TX are also carrying out an NSF funded Food-Energy-Water project to develop a model to optimize the resources allocation and to support decision making processes. The project involves many stakeholders from agriculture, water and energy users and agencies.
CA will continue examining the effects of global climate change on water resources availability in the Sierra Nevadas. A representative headwater basin near the headwaters of the San Joaquin River, with natural flow in the upper watershed, was selected for hydrologic simulations using the HSPF model and climate change scenarios. Field trials will also determine BMPs for irrigating with degraded waters and applying pesticides.
CA in collaboration with MN will extend the capabilities of the computationally efficient semi distributed hydrologic modeling framework, Soil Moisture And Runoff simulation Toolkit (SMART) (Ajami et al., 2016), for large catchment scale simulations. CA is developing a website-based salinity management tool for the growers to guide soil salinity leaching aiming at reducing water use and nitrate leaching in irrigated cropland.
ND and MN will study the vadose–saturated zone continuum to develop nutrient management methods that reduce loading to and degradation of groundwater and subsurface tile drainage, while maintaining optimal economic benefits. MN and potentially CA will develop the ability to quantify the water fluxes across the surfaces of land masses of arbitrary size, providing the basis for developing new brands of hydrologic and transport models that can incorporate flux calculating procedures into SWAT or similar models.
WA, in collaboration with the USDA NSERL and US Forest Service Rocky Mountain Research Station, will continue to improve, test, and apply the WEPP (Water Erosion Prediction Project) model for watershed assessment and management. OK will work to provide surface water managers with improved seasonal streamflow forecasts that are useful in making water planning decisions, particularly in the context of reservoir management. At the heart of these prediction systems will be calibrated and validated models of streamflow, which incorporate soil moisture data to produce probabilistic, quantitative forecasts of the potential streamflow totals for the upcoming season.
KY will continue examining a field scale approach to water and solute transport processes which is based on scale-dependent spatial distribution of treatments. This design allows quantifying transport processes in heterogeneous field soils (Wendroth et al., 2011; Yang et al., 2013; Yang and Wendroth, 2014).
TX and VA will conduct remote- and field-based studies to assess impacts to landscapes (e.g., soil, water and ecosystem resources) from above-ground activities, including urbanization, energy development and land conversion. VA will use a comprehensive dataset from 11 U.S. cities to assess effects of urbanization on soil hydrological function.
KS will study the upscaling and downscaling of surface and root-zone soil moisture at the field and landscape level by combining soil moisture observations from the Kansas mesonet network, a COSMOS rover, and satellite missions. The goal is to generate a high-resolution map of root-zone soil moisture storage to better assess drought conditions in the state of Kansas.
Measurement of Progress and Results
Outputs
- ● New methods and approaches to study mass and energy transport processes in soils at spatial and temporal scales appropriate for effective resource management. Comments: Information on the scientific advancements, research findings, and inter-institutional collaborations throughout the world will continue to be provided through the committee web site maintained at NDSU. This project will engage scientists and provide answers on short-term problems affecting US agriculture and environmental protection in the areas of salinity, water quality, solute transport, evapotranspiration, soil water and chemical transport properties and other areas, especially ecological processes. Our research will focus on long-term problems, such as identifying and characterizing the dominant processes affecting the transport of mass and energy through soils and other porous media at various management scales. This project is unique among multistate committee efforts because, rather than collaborating on a single focused objective, many collaborative projects are conducted simultaneously by organized groups of participating members and others. These extensive collaborations are established and maintained through our organizational structure. This strategy is inevitable given the diversity of problems addressed, but is also highly desirable, as information gained from the specific collaborations are shared with global science communities.
- New knowledge affecting the environmental impacts of soil, water and chemically-based agricultural practices and broader land uses. Comments: See above comment.
- Improved numerical modeling capabilities from advances in existing models, e.g., multi-dimensional Hydrus model. Comments: See above comment.
- Methods to transfer results from non-destructive imaging into quantitative assessments of soil structure. Comments: See above comment.
- New instruments and analytical techniques for measuring water, chemical, and energy fluxes. Comments: See above comment.
- New tools and capabilities to quantify and monitor movement of agricultural contaminants from the vadose zone to ground water and to the atmosphere. Comments: See above comment.
- New methodologies (computer and analytical models) that integrate knowledge of mass and energy transport, improving resource management. Comments: See above comment.
- Plant growth media for reduced gravity environments of space. Comments: See above comment.
- Sensitivity assessments of drip irrigation management scenarios to assist farmers and managers as they adoption sustainable and efficient irrigation systems. Comments: See above comment.
- New thermal instruments and analytical methods for calculating sensible and latent heat fluxes including approaches to separate latent heat flux arising from evaporation and transpiration. Comments: See above comment.
- New statistical methods to link crop yield and variability from sensor measurements. Comments: See above comment.
- Updated versions of various numerical tools being developed: HYDRUS, HP1, CW2D module, the UNSATCHEM modules, the HYDRUS package for MODFLOW, SMART, and others. Comments: See above comment.
- New collaborations with microbiologists, ecologists, hydrologists, pedologists, and engineers who are predicting landscape responses from land use/land cover changes and climate variability. Comments: See above comment.
- Machine learning methods and tools to interpret large datasets from field studies and monitoring sites. Comments: See above comment.
- Further develop fiber optic DTS as a reliable soil moisture sensor. Comments: See above comment.
- Continued support of young faculty, postdocs and students, who are dedicated to studying the role of soil physics in environmental processes. Comments: See above comment.
- New graduate level, international course on soils in the global groundwater-agriculture interface. Comments: See above comment.
- New international collaborations, like the International Soil Modeling Consortium, that tie together many of the activities discussed herein. Comments: See above comment.
Outcomes or Projected Impacts
- New scientific knowledge and information about fundamental physical, chemical and biological processes will help to understand the transformation and transport of pesticides, pathogens, colloids, nutrients, salts, and emerging contaminants. The breadth of research topical areas will lead to a diverse set of outcomes that will significantly improve our knowledge regarding mass and energy transport in near-surface environments, and thereby improve the ability to determine how to enhance soil sustainability.
- Results will quantify the amount, fate, and transport of bioactive compounds from commercial manure handling and disposal methods.
- Improved understanding of the role of scale in basin-scale processes, including evapotranspiration, water balance and ecological functions and services.
- Improved understanding of processes that control behavior of emerging contaminants from gray water or treated wastewater in soil/water systems, including mitigation practices.
- Examine herbicide leaching to groundwater versus metabolism in the soil and in plant in diverse climates.
- Evaluate impacts of plastics in soils and fate and transport of nano- and microplastics in soils.
- Guidance to producers on the sustainability of drip irrigation in salt-affected soils with reduced quantity or marginal quality irrigation water will be improved.
- Develop and demonstrate simple and reliable soil water sensing systems to accurately quantify soil water balance and other hydrological processes.
- Broader use of frequency-dependent dielectric measurements in soil to infer soil textural properties, in addition to water content and electrical conductivity.
- Improved measurement techniques will better characterize the relationships between soil, climate, and geomorphic position at the landscape scale.
- Improvements in the evaluation and prediction of land-use changes on managed lands (impact of grazing on compaction, erodibility, plant communities).
- Landscape-scale predictive capabilities for soil evaporation implemented into large-scale climate models.
- Improved protection of soil and water resources from energy production (i.e., coal and mineral extraction, in particular mine tailings).
- Stronger connections developed between atmospheric measurements and soil physical and hydraulic properties, especially under climate change scenarios, will improve how soil processes are embedded into atmospheric models.
- Solution to the closure problem for various hydrologic fluxes, including heterogeneity adopted into lumped parameter models (i.e., SWAT).
- Establish statistical structure between soil water, soil C storage and soil gas (C, N) emission fluxes in different land use systems before and after land use transition.
- Assessments, briefings, and legislative testimony in direct support of policy and decision-making bodies at the state and federal level.
Milestones
(2019):Complete research on delineating short-term influence of tillage on water storage and crop yield. Model testing of methane fate and transport in grassland soil. Complete drip-irrigation experiments in salt-affected soil. Conduct novel evaporation experiments using an environmentally-controlled laboratory lysimeter and large weighing lysimeters. Apply numerical modeling to evaluate optimal plant growth media for reduced gravity environments. Characterize and present ET estimates of montane vegetation in stony soils of the Intermountain West and agricultural systems in the Great Plains. Develop methods to extract and quantify micro- and nanoplastics in soils. Complete soil moisture balance calculations using field monitoring data to characterize soil moisture storage-deep percolation relations. Evaluate novel water content sensor-based net water flux algorithm on irrigated-Texas and arid-Nevada soil lysimeters. Evaluate node and gateway system for soil water sensor data transmission to the Cloud in the US, Jordan and Uzbekistan. Evaluate effectiveness of deep (2.3 m) profiles of TDR sensors (15) to determine hourly and daily change in soil water storage in a large weighing lysimeter. Conduct research on a soil fracking device utilized to increase permeability beneath clogged or poorly performing rain gardens. Complete research on the effect of utilizing either the Type II or Atlas 14 intensity nested hyetographs as a basis to model infiltration and runoff. Evaluate stable isotope partitioning within soils during various levels of non-equilibrium flow. Assess nitrogen profiles in a heterogeneous vadose zone underneath an almond orchard. Design and test a new, relatively large thermo-TDR sensor that provides more accurate soil water content and thermal property values then do the existing thermo-TDR sensors.(2020):Complete experiments to examine the impact of imposed soil water deficits on root elongation. Model testing of methane fate and transport in forest ecosystems. Conduct lysimeter studies to investigate water use and redistribution of plant communities in the desert. Improve segmentation and quantitative analysis methods for X-Ray CT images. Develop water content sensor standards for electromagnetic-based sensors. Complete studies on the potential for water flow and contaminant transport from energy (coal) production. Apply REW concept to the SMART model platform, and apply the model to a select watershed in Minnesota. Complete quantification of field to large basin scale transfer of nitrogen and salts in irrigated agricultural landscape through soils to groundwater. Present results from prototype Thermo-TDR intended for commercialization. Complete evaluation of node-gateway-Cloud system for soil water sensing and integrate system into variable rate irrigation scheduling and control system. Complete research on alternative design hyetographs that yield more realistic precipitation intensities and resulting infiltration and runoff. Complete research and model development to quantify role of preferential flow during transport of pesticides and antibiotics. Complete research on modeling coupled soil structural dynamics and flow and transport processes. Establish a combined soil hydraulic property, thermal property, and electrical property dataset for a range of soil conditions. Conduct analysis on interfacial properties of biofilms as they age. Develop methods for quantitative extraction of micro- and nanoplastics from soils. Determine water activities in Atacama desert samples and relate to microbial life to determine dry limits of life on Earth.
(2021):Complete research on delineating short-term influence of tillage on water storage and crop yield. Model testing of methane fate and transport in desert ecosystems. Derive a physically-based model that relates soil properties, topology, and atmospheric conditions to soil evaporation rates. Design soil surface preparation strategies for agricultural and environmental management, to reduce evaporation rates. Develop benchmarks for electromagnetic sensor quality standards testing. Combine measurements of water content, water potential, and soil bulk electrical conductivity at the landscape scale. Partition herbicides that leach to groundwater versus metabolism in soil and plants. Couple landscape scale water balance with meso-scale climate modeling. Demonstrate models describing frequency-domain complex dielectric permittivity of soils and porous media. Complete evaluation of deep TDR sensor profiles for determination of soil water change in storage compared with large weighing lysimetry. Complete assessment of three-year high efficiency fertigation in almond orchards with vadose zone monitoring network. Develop and test a numerical heat and water transfer model that accounts for soil thermal and hydraulic properties as a function of soil bulk density and water content. Conduct analysis of changes caused by natural abundance biofilm on the moisture retention curve for sandy soil. Quantify interactions between plastic particles and plant roots and soil fauna.
(2022):Complete lysimeter measurements and analyses of nutrient movement toward ground water. Provide design criteria for vegetative covers of tailings impoundments and landfills in semiarid and arid environments. Code and distribute software package for segmenting and analyzing X-ray CT images. Complete experiments to examine temporal and spatial distribution of soil C and N dynamics and greenhouse gas emissions. Implement approved water content sensor standards through ASTM as a benchmark for sensor quality. Complete analyses of hydromechanical and hydraulic property impacts from expanding root structure in soils. Develop improved sensors and scaling relationships for physical property characterization and process determination. Modeling assessment of nitrate fate and transport through heterogeneous unsaturated zones in irrigated permanent crops. During the annual multistate project meeting, identify a team of member volunteers to lead the new proposal writing efforts for next five years.
(2023):Review soil water sensing systems for water balance determinations. Review and ensure effectiveness of commercialization efforts. Complete experiments to examine the impact of imposed soil water deficits on root elongation. Incorporate colloidal processes into workable numerical approaches. Quantify fate and transport of bioactive compounds from manure operators. Develop strategies to reduce greenhouse gas emissions from agricultural soils. Link atmospheric measurements and PTF-derived soil hydraulic properties to predict soil water resources. Incorporate new physically-based modeling methods for simulating watershed-scale processes. Develop a physically-based conceptual model of feedback mechanisms that affect C storage and release. Describe dynamic behavior of water and soil gas emission fluxes during and after land use transition. Strive to make sensors and algorithms developed by this group commercially available. Complete BMPs for irrigating with degraded water to reduce air contamination from ag pesticides. Assess nitrate flushing in heterogeneous vadose zones under agricultural managed aquifer recharge conditions. Develop and test a method to characterize soil solid phase structure and soil pore structure based on soil hydraulic property, thermal property, and electrical property measurements. Complete soil health assessments to quantify effects of plastics in soil ecosystems. Prepare and submit new proposal for 5-year project renewal.
Projected Participation
View Appendix E: ParticipationOutreach Plan
The project members comprise a group of dedicated soil, water and environmental scientists and engineers who excel in the communication of their research through different communications platforms, and who are active participants in soil and environmental research at universities and federal facilities across the country. They also lead undergraduate and graduate education and supervise research. Many of our members conduct workshops, short courses, and classes to educate other scientists and the public, contribute to state, regional and federal agencies. Although most of our members do not have formal extension appointments, our members regularly participate in field days organized by extension faculty at the land grant universities. Our members are involved in publishing extension pamphlets, articles, and videos on their research projects. We are also involved in providing inputs to federal regulations pertinent to our research activities. For instance, our work on environmental fate of microplastics helps the National Organic Standards Board to determine how to regulate the use of plastics in organic agriculture. Members of our group are actively involved in these discussion. As another instance, results of hydrologic and water quality modeling in agricultural production settings in southeastern Minnesota is being used to guide placement of nitrogen management BMPs intended to reduce nitrate transport to groundwater and surface water in the region. W3188 members have published their findings in top-tier, peer-reviewed journals, targeting both science and engineering communities and are actively involved in organizing and participating many professional society international/national/regional meetings (SSSA, AGU, ESA, EGU, ASABE, ASCE, GRA, GSA), and major workshops and symposia sponsored by these societies. They serve as Editors and Associate Editors on journal editorial boards and as ad hoc manuscript reviewers, and therefore, enhance the overall quality of published research. Members also serve the scientific community by their engagement in competitive grant review panels of federal and regional entities, and as peer reviewers for domestic and international grant proposals. Our members have been instrumental in creating the International Soil Modeling Consortium, now with more than 600 members worldwide. They frequently engage with legislators at the state and federal level, and with managers, directors, and personnel in local, regional, state, and national water management organizations (e.g., irrigation and water districts, state agencies, regional MOUs) to support scientifically-based policy development and assist in technically sound decision-making. Through entrepreneurship, committee members have developed commercially available instruments, analytical tools, and textbooks. We fully expect this type of outreach to continue and thrive. Results of our work will be available through the annual project report, the project website ((https://www.nimss.org/projects/view/mrp/outline/16636), periodic joint meetings with related multistate research and/or coordinating groups, and through the international reputations and professional visibility of participants. The members will also work with consulting firms, companies and farmers to adopt measurement and management technologies.
Organization/Governance
The current W3188 multistate committee consists of members representing SAESs, other Universities, the USDA-ARS, National Laboratories, and other research units. In addition, visiting scientists (U.S. and global) participate along with member hosts. Officers of the new W4188 will be the Chair and Secretary. The Secretary is elected each year at the annual meeting and advances to Chair the following year. The Chair may appoint members to serve on subcommittees as needed.
Meetings will be approved by the AA. The current Secretary will be responsible for making local arrangements. Committee meetings typically have been held in Las Vegas, NV during early January, but members may decide (by voting) to choose new locations (the 2019 meeting was held in Riverside, CA). At each meeting, research accomplishments are reviewed, new opportunities and recommendations for multistate coordination/collaboration are discussed, and strategies for maximizing the impact of committee productivity are suggested. In addition, we invite scientists from different disciplines (geomorphology, land use planning, ecology) to provide opportunities for initiating transdisciplinary collaboration on new cutting-edge research directions that would engage areas of our expertise. In this way, fresh perspectives are injected into the committee, encouraging outward-looking and multi-disciplinary approaches toward pressing agricultural and environmental problems. The project committee and its precursors have had strong historical participation at the annual meetings (35-40 attendees, 75 in 2019), with new members inducted each year to ensure longevity and infusion of fresh perspectives. Although meeting attendance can vary from year to year, existing W3188 members have indicated a strong desire to continue participation.
Literature Cited
Adhikari P., M.K. Shukla and J. Mexal. 2012. Spatial variability of Infiltration rate and sodium content of Desert soils: Implications for management of irrigation using treated wastewater. Transactions of ASABE. 55(5): 1711-1721.
Ajami, H., U. Khan, N.K. Tuteja, A. Sharma. 2016. Development of a Computationally Efficient Semi-distributed Hydrologic Modeling Application for Soil Moisture, Lateral Flow and Runoff Simulation, Environmental Modelling & Software. doi: 10.1016/j.envsoft.2016.09.002
Aminzadeh, B., and D.A. DiCarlo. 2010. The transition between sharp and diffusive wetting fronts as a function of imbibing fluid properties, Vadose Zone Journal, 9:588-596, doi:10.2136/vzj2009.0072
Appleman, T.D., Higgins, C.P., Quiñones, O., Vanderford, B.J., Kolstad, C., Zeigler-Holady, J.C., Dickenson, E.R.V., 2014. Treatment of poly- and perfluoroalkyl substances in U.S. full-scale water treatment systems. Water Research 51, 246-255.
Arora, B., B.P. Mohanty, J.T. McGuire, and I. Cozzarelli, 2013, Temporal Dynamics of Biogeochemical Processes at the Norman Landfill Site. Water Resources Research. 49, doi: 10.1002/wrcr.20484, 2013.
Baird, A.J., and R.L. Wilby. 1999. Eco-hydrology: Plants and Water in Terrestrial and Aquatic Environments Routledge, London.
Barzegar, R., A. A. Moghaddam, R. Deo, E. Fijani, and E. Tziritis, 2018. Mapping groundwater contamination risk of multiple aquifers using multi-model ensemble of machine learning algorithms, Sci. Total Environ., vol. 621, pp. 697–712.
Baver, L.D. 1940. Soil Physics, John Wiley & Sons, New York.
Bihannic, I., Tchoubar, D., Lyonnard, S., Besson, G., Thomas, F. 2001. X-ray scattering investigation of swelling clay fabric 1. The dry state. Journal of Colloid Interface Science 240(1), 211-218.
Bossi, R., Strand, J., Sortkjær, O., Larsen, M.M., 2008. Perfluoroalkyl compounds in Danish wastewater treatment plants and aquatic environments. Environment International 34(4), 443-450.
Bradford, S.A., and S. Torkzaban. 2008. Colloid transport and retention in unsaturated porous media: a review of interface-, collector-, and pore-scale processes and models. Vadose Zone J. 2008 7:667-681.
Bradford, S.A., Šimůnek, J., Bettahar, M., van Genuchten, M.T., S.R., Yates. 2003. Modeling colloid attachment, straining, and exclusion in saturated porous media. Environ. Sci. Technol. 37:2242-2250.
Cahill, A.T., F. Ungaro, M.B. Parlange, M. Mata, and D.R. Nielsen. 1999. Combined spatial and Kalman filter estimation of optimal soil hydraulic properties. Water Resour. Res. 35:1079-1088.
Cai, G., J. Vanderborght, V. Couvreur, C.M. Mboh, and H. Vereecken. 2017. Parameterization of root water uptake models considering dynamic root distributions and water uptake compensation. Vadose Zone J. 17:160125. doi:10.2136/vzj2016.12.0125
Carminati, A., A. B. Moradi, D. Vetterlein, P. Vontobel, E. Lehmann, U. Weller, H.-J¨org Vogel and S. E. Oswald. 2010. Dynamics of soil water content in the rhizosphere. Plant Soil 332:163–176. DOI 10.1007/s11104-010-0283-8
Carminati, A. 2013. Rhizosphere wettability decreases with root age: a problem or a strategy to increase water uptake of young roots? Frontiers in Plant Sci. Vol. 4, doi: 0.3389/fpls.2013.00298
Campbell, G.S. 1977. An Introduction to Environmental Biophysics. 1st ed. Springer-Verlag, New York.
Campbell, G.S., and J.M. Norman. 1998. An Introduction to Environmental Biophysics. 2nd ed. Springer-Verlag, New York.
Chou, J.S., C. C. Ho, and H. S. Hoang, 2018. Determining quality of water in reservoir using machine learning, Ecol. Inform., vol. 44, no. January, pp. 57–75.
Clausnitzer, V., and J.W. Hopmans. 2000. Pore-scale measurements of solute breakthrough using microfocus X-ray computed tomography. Water Resour. Res., 36:2067-2079.
Comegna, V., and C. Vitale. 1993. Space-time analysis of water status in a volcanic Vesuvian soil. Geoderma 60:135-158.
de la Mota Daniel, F. J., Day, S. D., Owen, J. S., Stewart, R. D., Steele, M. K., & Sridhar, V. 2018. Porous-permeable pavements promote growth and establishment and modify root depth distribution of Platanus × acerifolia (Aiton) Willd. in simulated urban tree pits. Urban Forestry & Urban Greening, 33, 27-36. doi:10.1016/j.ufug.2018.05.003
Deb, S. K., M. K. Shukla, J. Šimůnek, and J. G. Mexal. 2013. Evaluation of spatial and temporal root water uptake patterns of a flood-irrigated pecan tree using the HYDRUS (2D/3D) model. ASCE, Irrigation and Drainage Engineering. 139: 599-611.
Deb S., P. Sharma, M.K. Shukla, and J. Simunek. 2015. Numerical Evaluation of Nitrate Distributions in the Onion Root Zone under Conventional Furrow Fertigation. Journal of Hydrologic Engineering ASCE. DOI: 10.1061/(ASCE)HE.1943-5584.0001304.
DeBano, L. 2000. The role of fire and soil heating on water repellency in wildland environments: a review. Journal of Hydrology, 231-232:195-206.
deVries D.A. 1963. Thermal properties of soils. In Physics of Plant Environment, van Wijk W.R., (ed.), Wiley: New York, 210-235.
Duliu, O.G. 1999. Computer axial tomography in geosciences: an overview. Earth Science Reviews 48: 265-281.
Ebel, B.A., and J.A. Moody. 2016. Synthesis of soil-hydraulic properties and infiltration timescales in wildfire-affected soils. Hydrological Processes 2017, 31:324-340. http://dx.doi.org/10.1002/hyp.10998.
Ellsworth, T.R., and C.W. Boast. 1996. Spatial structure of solute transport variability in an unsaturated field soil. Soil Sci. Soc. Am. J. 60:1355-1367.
Ewing, R.P, and R. Horton. 2007. Thermal conductivity of a cubic lattice of spheres with capillary bridges, J. Phys. D: Appl. Phys. 40:4959-4965.
Fan, Z., F.X.M. Casey, G.L. Larsen, and H. Hakk. 2007a. Persistence and fate of 17β-estradiol and testosterone in agricultural soils. Chemosphere 67:886-895.
Fan, Z., Casey, F.X.M., H. Hakk, and G.L. Larsen. 2007b. Discerning and modeling the fate and transport of testosterone in undisturbed soil. J. Environ. Qual. 36:864-873.
Flores A., M.K. Shukla, D. Daniel, A. Ulery, B. Schutte, G. Pichionni and S. Fernald. 2016. Evapotranspiration Changes with Irrigation Using Saline Groundwater and RO Concentrate. J. Arid Environments. 131:35-45
Freeze, R.A., 1970. Three-dimensional, transient, saturated-unsaturated flow in a groundwater basin, Water Resour. Res., 7(2): 347-366.
Gardea-Torresdey, J.L., Rico, C.M., White, J.C., 2014. Trophic transfer, transformation, and impact of engineered nanomaterials in terrestrial environments. Environmental Science & Technology 48(5), 2526-2540.
Gaur, N., and B.P. Mohanty, 2013. Evolution of Physical Controls for Soil Moisture in Humid and Sub-Humid Watersheds, Water Resources Research, 49, 1-15, doi:10.1002/wrcr.20069.
Gogos, A., Knauer, K., Bucheli, T.D., 2012. Nanomaterials in plant protection and fertilization: Current state, foreseen applications, and research priorities. Journal of Agricultural and Food Chemistry 60(39), 9781-9792.
Goh, G.B., N. O. Hodas, and A. Vishnu, 2017. Deep learning for computational chemistry, J. Comput. Chem., vol. 38, no. 16, pp. 1291–1307.
Gottschalk, F., Sonderer, T., Scholz, R.W., Nowack, B., 2009. Modeled environmental concentrations of engineered nanomaterials (TiO2, ZnO, Ag, CNT, Fullerenes) for different regions. Environmental Science & Technology 43(24), 9216-9222.
Gottschalk, F., Sonderer, T., Scholz, R.W., Nowack, B., 2010. Possibilities and limitations of modeling environmental exposure to engineered nanomaterials by probabilistic material flow analysis. Environmental Toxicology and Chemistry 29(5), 1036-1048.
Green, T., B.C. Bates, S.P. Charles, and P.M. Fleming. 2007. Physically-based simulation of potential effects of carbon dioxide-altered climates on groundwater recharge. Vadose Zone J. 6:597-609.
Green, T.R., G.H. Dunn, R.H. Erskine, J.D. Salas, and L.R. Ahuja. 2009. Fractal analysis of steady infiltration and terrain on an undulating agricultural field. Vadose Zone J. (in press).
Greenspan, H., B. van Ginneken, and R. M. Summers, 2016. Guest Editorial Deep Learning in Medical Imaging: Overview and Future Promise of an Exciting New Technique, IEEE Trans. Med. Imaging, 35(5), 1153–1159.
Greminger, P.J., K. Sud, and D.R. Nielsen. 1985. Spatial variability of field-measured soil-water characteristics. Soil Sci. Soc. Am. J. 49:1075-1082.
Guan, S., Dou, S., Chen, G., Wang, G., & Zhuang, J, 2015. Isotopic characterization of sequestration and transformation of plant residue carbon in relation to soil aggregation dynamics. Applied Soil Ecology 96, 18-24
Guelfo, J.L., Adamson, D.T., 2018. Evaluation of a national data set for insights into sources, composition, and concentrations of per- and polyfluoroalkyl substances (PFASs) in U.S. drinking water. Environ Pollut 236, 505-513.
Haghverdi, A., Öztürk, H. S., & Durner, W. 2018. Measurement and Estimation of the Soil Water Retention Curve Using Evaporation Method and Pseudo Continuous Pedotransfer Function. Journal of Hydrology. 563: 251-259.
Hallema, D.W., G. Sun, P.V. Caldwell, S.P. Norman, E.C. Cohen, Y. Liu, K.D. Bladon, and S.G. McNulty. 2018. Burned forests impact water supplies. Nature Communications 9, 1307, DOI: 10.1038/s41467-018-03735-6.
Hansen, D.J., J.T. McGuire, and B. P. Mohanty, 2011. Enhanced Biogeochemical Cycling and Subsequent Reduction of Hydraulic Conductivity Associated with Soil Interfaces in the Vadose Zone. Journal of Environmental Quality. 40, 40:1941–1954.
Hartmann, A., J. Šimůnek, M.K. Aidoo, S.J. Seidel, and N. Lazarovitch. 2017. Implementation and application of a root growth module in HYDRUS. Vadose Zone J. 17:170040. doi:10.2136/vzj2017.02.0040
He, H., M.F. Dyck, R. Horton, T. Ren, K.L. Bristow, J. Lv, and B. Si. 2018. Development and application of the heat pulse method for soil physical measurements. Reviews of Geophysics. 56. doi.org/10.1029/2017RG000584
Heitman, J.L., R. Horton, T. J. Sauer, and T. M. Desutter. 2008. Sensible heat observations reveal soil-water evaporation dynamics. J. Hydrometeorology, DOI: 10.1175/2007JHM963.1
Hemminga, M.A. and P. Buurman. 1997. NMR in soil science. Geoderma 80: 221-464.
Hopmans, J.W. 2006. Plant water and nutrient uptake in soil-root systems. 5.1. Rhizosphere Models, p. 495-496 Handbook of Methods used in Rhizosphere Research. Swiss Federal Research Institute WSL, Birmensdorf.
Hopmans, J.W., T. Vogel, and P.D. Koblik. 1992. X-ray tomography of soil water distribution in one-step outflow experiments. Soil Sci. Soc. Am. J., 56(2):355–362.
Hu, X-J, J-H Du, S-Y Lei and B-X Wang. 2001. A model for the thermal conductivity of unconsolidated porous media based on capillary pressure–saturation relation. Int. J. Heat Mass Transfer. 44:247-251.
Hu, X.C., Andrews, D.Q., Lindstrom, A.B., Bruton, T.A., Schaider, L.A., Grandjean, P., Lohmann, R., Carignan, C.C., Blum, A., Balan, S.A., Higgins, C.P., Sunderland, E.M., 2016. Detection of poly- and perfluoroalkyl substances (PFASs) in U.S. drinking water linked to industrial sites, military fire training areas, and wastewater treatment plants. Environmental Science & Technology Letters 3(10), 344-350.
Hubbert, K.R., H.K. Preisler, P.M. Wohlgemuth, R.C. Graham, and M.G. Narog. 2006. Prescribed burning effects on soil physical properties and soil water repellency in a steep chaparral watershed, southern California, USA. Geoderma, 130:284-298.
Hunt, A.G. and R.P Ewing. 2009. Percolation theory for transport in porous media. Lecture Notes in Physics 771, Springer, Berlin.
Hurley, S., Houtz, E., Goldberg, D., Wang, M., Park, J.-S., Nelson, D.O., Reynolds, P., Bernstein, L., Anton-Culver, H., Horn-Ross, P., Petreas, M., 2016. Preliminary Associations between the Detection of Perfluoroalkyl Acids (PFAAs) in Drinking Water and Serum Concentrations in a Sample of California Women. Environmental Science & Technology Letters 3(7), 264-269.
Ines, A.V.M., B.P. Mohanty, and Y. Shin, 2013. An Unmixing Algorithm for Remotely Sensed Soil Moisture, Water Resources Research. 49, 408–425, doi:10.1029/2012WR012379.
Isaaks, E.H. and R.M. Srivastava. 1989. Applied Geostatistics. Oxford University Press, New York.
Jones, S.B. and K. Shenai. 2007. Subsurface measurement needs for ecological, hydrological and agricultural applications, Circuits and Systems. MWSCAS. 50th Midwest Symposium. 5-8 Aug. 2007. pp. 754-757.
Kah, M., Beulke, S., Tiede, K., Hofmann, T., 2013. Nanopesticides: State of knowledge, environmental fate, and exposure modelling. Critical Reviews in Environmental Science and Technology 43(16), 1823-1867.
Kah, M., Hofmann, T., 2014. Nanopesticide research: Current trends and future priorities. Environment International 63(0), 224-235.
Karpatne, A., W. Watkins, and J. Read, and V. Kumar, 2018. Physics-guided Neural Networks ( PGNN ): An Application in Lake Temperature Modeling, ACM SIGKDD, arXiv:1710.11431.
Ketcham, R.A., and W.D Carlson. 2001. Acquisition, optimization, and interpretation of X-ray computed tomographic imagery: Applications to the geosciences. Computers Geosciences. 27:381-400.
Kirkham, M.B. 2005. Principles of Soil and Plant Water Relations Elsevier, Burlington, MA.
Kollet, S.J., R.M. Maxwell, C.S. Woodward, S. Smith, J. Vanderborght, H. Vereecken, and C. Simmer, 2010. Proof of concept of regional scale hydrologic simulations, Water Resour. Res., 46: W04201, doi:10.1029/2009WR008730.
Kojima,Y., J.L. Heitman, M. Sakai, C. Kato, and R. Horton. 2018. Bulk density
effects on soil hydrologic and thermal characteristics: a numerical investigation.
Hydrological Processes. 32:2203-2216. DOI: 10.1002/hyp.13152
Kolpin, D.W., E.T. Furlong, M.T. Meyer, E.M. Thurman, S.D. Zaugg, L.B. Barber, and H.T. Buxton. 2002. Pharmaceuticals, hormones, and other organic wastewater contaminants in U.S. streams, 1999-2000: A national reconnaissance. Environ. Sci. Technol. 36, 1202 -1211.
Kubiëna, W.L. 1938. Micropedology, Collegiate Press Inc., Ames, IA.
Kumar, K., C. Gupta, S., Chander, Y., Singh, A.K., 2005. Antibiotic use in agriculture and its impact on the terrestrial environment. Advances in Agronomy. Donald, L.S. (ed), pp. 1-54, Academic Press, San Diego, CA.Larue, C., Castillo-Michel, H., Sobanska, S., Cécillon, L., Bureau, S., Barthès, V., Ouerdane, L., Carrière, M., Sarret, G., 2014. Foliar exposure of the crop Lactuca sativa to silver nanoparticles: Evidence for internalization and changes in Ag speciation. Journal of Hazardous Materials 264(0), 98-106.
Lazarovitch, N., J. Vanderborght, Y. Jin, and M.Th. van Genuchten. 2018. The root zone: Soil physics and beyond. Vadose Zone J. 17:180002. doi:10.2136/vzj2018.01.0 0 02
Lebron, I., M.D. Madsen, D.G. Chandler, D.A. Robinson, O. Wendroth, and J. Belnap. 2007. Ecohydrological controls on soil moisture and hydraulic conductivity within a pinyon-juniper woodland. Water Resour. Res. 43.
Lee, L.S., Carmosini, N., Sassman, S.A., Dion, H.M., Sepúlveda, M.S., 2007. Agricultural contributions of antimicrobials and hormones on soil and water quality. Advances in Agronomy. Donald, L.S. (ed), pp. 1-68, Academic Press.
Li, H., C. Pan, and C. T. Miller. 2005. Pore-scale investigation of viscous coupling effects for two-phase flow in porous media. Phys. Rev. E., 72, 026705.
Li, Q., Chen, X-J., Chen, X., Zhuang, J, 2019. Cadmium removal from soil by fulvic acid-aided hydroxyapatite nanofluid. Chemosphere 215, 227-233
Liang, X., Radosevich, M., Löffler, F., Schaeffer, S., and Zhuang, J. 2019. Impact of microbial iron oxide reduction on the transport of diffusible tracers and non-diffusible nanoparticles in soils. Chemosphere 220, 391-402.
Liu, G., Y. Lu, M. Wen, T. Ren, and R. Horton. 2017. Advances in the heat-pulse
technique: improvements in measuring soil thermal properties. Methods of Soil
Analysis Vol. 2, doi:10.2136/msa2015.0028.
Liu, Y., Racah, E., Prabhat, Correa, J., Khosrowshahi, A., Lavers, D., Kunkel, K., Wehner, M., Collins, W., 2016. Application of Deep Convolutional Neural Networks for Detecting Extreme Weather in Climate Datasets, arXiv preprint arXiv:1605.01156.
Logsdon, S.D., R. Horn and M. Berli, 2013. Quantifying and Modeling Soil Structure Dynamics. Advances in Agricultural Systems Modeling Series. Soil Science Society of America, Madison, WI.
Lu, Y., R. Horton, and T. Ren. 2018. Simultaneous determination of soil bulk density
and water content: a heat pulse‐based method. European Journal of Soil Science
69:947-952. doi: 10.1111/ejss.12690
Lu, Y., S. Lu, R. Horton, and T. Ren. 2014. An empirical model for estimating soil thermal conductivity from texture, water content, and bulk density. Soil Science Society of America Journal 78:1859-1868.
Ma, X., Geiser-Lee, J., Deng, Y., Kolmakov, A., 2010. Interactions between engineered nanoparticles (ENPs) and plants: Phytotoxicity, uptake and accumulation. Science of the Total Environment 408(16), 3053-3061.
Malchi, T., Maor, Y., Tadmor, G., Shenker, M., Chefetz, B., 2014. Irrigation of root vegetables with treated wastewater: Evaluating uptake of pharmaceuticals and the associated human health risks. Environmental Science & Technology 48(16), 9325-9333.
Marçais, J. and J. R. de Dreuzy, 2017. Prospective Interest of Deep Learning for Hydrological Inference, Groundwater, vol. 55, no. 5, pp. 688–692.
Marchant, B., & Arrouays, D., 2014. Current issues and applications of soil monitoring. European Journal of Soil Science, 65(6), 777-778. doi:10.1111/ejss.12200
McCarthy, J.F., Ilavsky, J., Mayer, L.M., Jastrow, J.D., Perfect, E., Zhuang, J. 2008. Protection of organic carbon in soil microaggregates occurs via restructuring of aggregate porosity and filling of pores with accumulating organic matter. Geochim Cosmochim. Acta 72, 4725-4744.
McGechan, M.B., and D.R. Lewis. 2002. Transport of particulate and colloid-sorbed contaminants through soil. Part 1: General principles. Biosyst. Engineer. 83, 255-273.
Miralles, P., Church, T.L., Harris, A.T., 2012. Toxicity, uptake, and translocation of engineered nanomaterials in vascular plants. Environmental Science & Technology 46(17), 9224-9239.
Moody, J.A., D.A. Kinner, and X. Ubeda. 2009. Linking hydraulic properties of fire-affected soils to infiltration and water repellency. Journal of Hydrology, 379(3-4):291-303. https://doi.org/10.1016/j.jhydrol.2009.10.015.
Moody, J., R.A. Shakesby, P.R. Robichaud, S.H. Cannon, and D.A. Martin. 2013. Current research issues related to post-wildfire runoff and erosion processes. Earth-Science Reviews, 122:10-37.
Nasta, P., N. Romano, S. Assouline, J.A. Vrugt and J. W. Hopmans. 2013. Prediction of spatial variable unsaturated hydraulic conductivity using scaled soil particle size distribution functions. Water Resour Res. 49:1-11. Doi:10.1002/wrcr.20255.
Nielsen, D.R. 1987. Emerging frontiers in soil science. Geoderma 40:267-273.
Nielsen, D.R. 1997. A challenging frontier in hydrology - The vadose zone. In: N. Buras (ed.) Reflections on hydrology: Science and practice. AGU, pp. 205-226.
Nielsen, D.R., J.W. Biggar, and K.T. Erh, 1973. Spatial variability of field-measured soil water properties. Hilgardia 42:215-259.
Nielsen, D.R., and O. Wendroth. 2003. Spatial and temporal statistics - sampling field soils and their vegetation. Catena, Reiskirchen, Germany. 416 p.
NRC, 2012. A research strategy for environmental, health, and safety aspects of engineered nanomaterials, Committee to Develop a Research Strategy for Environmental, Health, Safety Aspects of Engineered Nanomaterials, National Research Council (NRC), The National Academies Press.
Or, D., B.F. Smets, J.M. Wraith, A. Duchesne, and S.P. Friedman. 2007. Physical constraints affecting bacterial habitats and activity in unsaturated porous media - A review. Adv. Water Resour. 30:1505-1527.
Or, D., and M. Tuller, 1999. Liquid retention and interfacial area in variably saturated porous media: upscaling from single pore to sample scale model. Water Resour. Res., Vol.35, No.12, 3591-3606.
Oschner, T.E., M.H. Cosh, R.H. Cuenca, W.A. Dorigo, C.S. Draper, Y. Hagimoto, Y.H. Kerr, K.M. Larson, E.G. Njoku, E.E. Small, and M. Zreda, 2013. State of the art in large-scale soil moisture monitoring, Soil Sci. Soc. Am. J., doi:10.2136/sssaj2013.03.0093
Oswald, S.E., M. Menon, A. Carminati, P. Vontobel, E. Lehmann and R. Schulin. 2008. Quantitative imaging of infiltration, root growth, and root water uptake via neutron radiography. Vadose Zone Journal 7: 1035-1047. doi:10.2136/vzj2007.0156.
Parise, M. and S.H. Cannon. 2012. Wildfire impacts on the processes that generate debris flows in burned watersheds. Natural Hazards, 61:217-227.
Perret, J., S.O. Prasher, A. Kantzas, K. Hamilton, and C. Langford. 2000. Preferential solute flow in intact soil columns measured by SPECT scanning. Soil Sci. Soc. Am. J. 64:469-477.
Qin, Q., Chen, X-J., and Zhuang, J. 2015. The fate and impact of pharmaceuticals and personal care products in agricultural soils irrigated with reclaimed water. Critical Reviews in Environ Sci. and Technol. 45 (13), 1379-1408.
Radlinski, A.P., Mastalerz, M., Hinde, A.L., Hainbuchner, M., Rauch, H., Baron, M., Lin, J.S., Fan, L., and Thiyagarajan, P. 2004. Application of SAXS and SANS in evaluation of porosity, pore size distribution and surface area of coal. International Journal of Coal Geology 59(3-4), 245-271.
Rasmussen, F.B. 2001. Different approaches to analysis of small angle scattering experiments on porous aluminum hydroxide. Colloid Surf. A: Physicochem. Engineer Aspects 187-188, 327-335.
Reggiani, P. and J. Schellekens. 2003. Modelling of hydrological responses: The representative elementary watershed approach as an alternative blueprint for watershed modeling. Hydrol Proc. 17: 3785-3789.
Reyes, J., O. Wendroth, C. Matocha, J. Zhu, W. Ren, and A.D. Karathanasis. 2018. Reliably mapping clay content coregionalized with electrical conductivity. Soil Sci. Soc. Am. J. 82:578-592. doi:10.2136/sssaj2017.09.0327.
Reynolds, J.F., D.M.S. Smith, E.F. Lambin, B.L. Turner, II, M. Mortimore, S.P.J. Batterbury, T.E. Downing, H. Dowlatabadi, R.J. Fernandez, J.E. Herrick, E. Huber-Sannwald, H. Jiang, R. Leemans, T. Lynam, F.T. Maestre, M. Ayarza, and B. Walker. 2007. Global desertification: Building a science for dryland development. Science 316:847-851.
Rico, C.M., Majumdar, S., Duarte-Gardea, M., Peralta-Videa, J.R., Gardea-Torresdey, J.L., 2011. Interaction of nanoparticles with edible plants and their possible implications in the food chain. Journal of Agricultural and Food Chemistry 59(8), 3485-3498.
Roberson EB. and Firestone MK. 1992. Relationship between desiccation and exopolysaccharide production in a soil Pseudomonas sp. Appl. Environ. Micro., 58(4):1284-1291.
Robinson D.A., C.S. Campbell, J.W. Hopmans, B.K. Hornbuckle, S.B. Jones, R. Knight, F. Ogden, J. Selker, and O. Wendroth. 2008a. Soil moisture measurement for ecological and hydrological watershed-scale observatories: A review. Vadose Zone J. 7:358-389 (doi:10.2136/vzj2007.0143).
Robinson, D.A., H. Abdu, S.B. Jones, M. Seyfried, I. Lebron, and R. Knight. 2008b. Eco-geophysical imaging of watershed-scale soil patterns links with plant community spatial patterns. Vadose Zone J. 7:1132-1138.
Rodriguez-Iturbe, I. 2000. Ecohydrology: A hydrologic perspective of climate-soil-vegetation dynamics. Water Resour. Res. 36: 3–9, DOI:10.1029/1999WR900210
Rogasik, H., J.W. Crawford, O. Wendroth, I.M. Young, M. Joschko, and K. Ritz. 1999. Discrimination of soil phase by dual energy X-ray tomography. Soil Sci. Soc. Am. J. 63:741-751.
Rosenzweig, R., Furman, A., & Shavit, U. 2013. A channel network model as a framework for characterizing variably saturated flow in biofilm-affected soils. Vadose Zone Journal, 12(2).
Radolinski, J., Wu, J., Xia, K., & Stewart, R., 2018. Transport of a neonicotinoid pesticide, thiamethoxam, from artificial seed coatings. Science of the Total Environment, 618, 561-568. doi:10.1016/j.scitotenv.2017.11.031
Roth, K. 1995. Steady state flow in an unsaturated, two-dimensional, macroscopically homogeneous, Miller-similar medium. Water Resour. Res. 31:2127-2140.
Russell, M.B. 1960. Some physical aspects of plant growth. Soil Sci. Soc. Am. J. 24:439-440.
Sadeghi, M., B. Ghanbarian, and R. Horton. 2018. Derivation of an explicit form of the percolation-based effective medium approximation for thermal conductivity of partially saturated soils. Water Resour. Res. 54.https://doi.org/10.1002/2017 WR021714.
Sajedi-Hosseini, F. Malekian, A., Choubin, B., Rahmati, O., Cipullo, S., Coulon, F., & Pradhan, B., 2018. A novel machine learning-based approach for the risk assessment of nitrate groundwater contamination, Sci. Total Environ., vol. 644, pp. 954–962.
Sastry, P.U., Sen, D., Mazumder, S., Chandrasekaran, K.S. 2000. Structural variations in lignite coal: a small angle X-ray scattering investigation. Solid State Communications 114(6), 329-333.
Sauvé, S., Desrosiers, M., 2014. A review of what is an emerging contaminant. Chemistry Central Journal 8(1), 15.
Schaap, M.G., F.J. Leij, and M. Th. Van Genuchten. 1998. Neural network analysis for hierarchical prediction of soil hydraulic properties. Soil Sci. Soc. Am. J. 62:847-855.
Schaap, M.G., F.J. Leij, and M.Th. van Genuchten. 2001. ROSETTA: A computer program for estimating soil hydraulic parameters with hierarchical pedotransfer functions. J. Hydrol. 251:163-176.
Schaap, M.G., M.L. Porter, B.S.B. Christensen, and D. Widenschild, 2007. Comparison of pressure-saturation characteristics derived from computed tomography and lattice Boltzmann simulations. Water Resour. Res., 43, W12S06, doi:10.1029/2006WR005730.
Schaumann, G. E., B. Braun, D. Kirchner, W. Rotard, U. Szewzyk, and E. Grohmann. 2007. Influence of biofilms on the water repellency of urban soil samples. Hydrol. Process. 21:2276-2284. DOI: 10.1002/hyp.6746
Schnaar, G., and M.L. Brusseau. 2005. Pore-scale characterization of organic immiscible-liquid morphology in natural porous media using synchrotron X-ray microtomography. Environ. Sci. Tech. 39:8403-8410.
Schnaar, G., and M.L. Brusseau. 2006a. Characterizing pore-scale configuration of organic immiscible liquid in multiphase systems with synchrotron X-ray microtomography. Vadose Zone J. 5:641-648.
Schnaar, G., and M.L. Brusseau. 2006b. Characterizing pore-scale dissolution of organic immiscible liquid in natural porous media using synchrotron X-ray microtomography. Environ. Sci. Techn. 40:6622-6629.
Shukla M.K., R. Lal, M. Ebinger and C. Meyer. 2006. Physical and chemical properties of soils under Pinon-Juniper-Oak canopies in a semiarid ecosystem in New Mexico. J. Arid Environ. 66:673-685.
Shafer, D.S., M.H. Young, S.F. Zitzer, T.G. Caldwell, and E.V. McDonald. 2007. Impacts of interrelated biotic and abiotic processes during the past 125,000 years of landscape evolution in the Northern Mojave Desert, Nevada, USA. J. Arid Environ. 69:633-657.
Shen, C., 2017. A trans-disciplinary review of deep learning research for water resources scientists, arXiv:1712.02162, pp. 1–66.
Shillito, R.M., M. Berli, T.A. Ghezzenei, and E. Kaminski. 2019. Infiltration into water-repellent sands: The role of sorptivity, presentation at 2019 Soil Science Society of America International Soils Meeting, January 6 – 9, 2019, San Diego, CA.
Shin, Y., B.P. Mohanty, and A.V.M. Ines, 2012. Soil Hydraulic Properties in One-Dimensional Layered Soil Profile Using Layer-Specific Soil Moisture Assimilation Scheme. Water Resources Research. 48, W06529, doi:10.1029/2010WR009581.
Shouse, P.J., W.B. Russel, D.S. Burden, H.M. Selim, J.B. Sisson, and M.Th. van Genuchten. 1995. Spatial variability of soil water retention functions in a silt loam soil. Soil Sci. 159:1-12.
Shumway, R.H., and D. Stoffer. 2000. Time series analysis and its applications. Springer, New York, 549pp. Simunek, J., and J.W. Hopmans. 2008. Modeling compensated root water and nutrient uptake. Ecol. Modeling. doi:10.1016/j.ecolmodel.2008.11.004 .
Smucker, A.J.M., and J.W. Hopmans. 2007. Soil biophysical contributions to hydrological processes in the vadose zone. Vadose Zone J. 6:267-268.
Song, X., G. Zhang, F. Liu, D. Li, Y. Zhao, and J. Yang, 2016. Modeling spatio-temporal distribution of soil moisture by deep learning-based cellular automata model, J. Arid Land, vol. 8, no. 5, pp. 734–748, 2016.
Spellberg, B., Hansen, G.R., Kar, A., Cordova, C.D., Price, L.B., Johnson, J.R., 2016. Antibiotic resistance in humans and animals.
Stewart, R. D., Abou Najm, M. R., Rupp, D. E., & Selker, J. S., 2016. Modeling multidomain hydraulic properties of shrink‐swell soils. Water Resources Research, 52(10), 7911-7930. doi:10.1002/2016WR019336
Stock, S.R. 1999. X-ray microtomography of materials. International Materials Reviews, 44(4):141-163.
Stockton, J.G., and A.W. Warrick. 1971. Spatial variability of unsaturated hydraulic conductivity. Soil Sci. Soc. Am. Proc. 35:847-848.
Tao, Y., X. Gao, K. Hsu, S. Sorooshian, and A. Ihler, 2016. A Deep Neural Network Modeling Framework to Reduce Bias in Satellite Precipitation Products, J. Hydrometeorol., vol. 17, no. 3, pp. 931–945.
Tian, Z., W. Gao, D. Kool, T. Ren, R. Horton, and J.L. Heitman. 2018a. Approaches
for estimating soil water retention curves at various bulk densities with the extended
van Genuchten model. Water Resour. Res. 10.1029/2018WR022871
Tian, Z., Y. Lu, T. Ren, R. Horton, J. L. Heitman. 2018b. Improved thermo-time
domain reflectometry method for continuous in-situ determination of soil bulk
density. Soil & Tillage Research 178:118–129. doi.org/10.1016/j.still.2017.12.021.
Tong, B., Z. Gao, R. Horton, Y. Li, and L. Wang. 2016. An empirical model for
estimating soil thermal conductivity from soil water content and porosity. J.
Hydrometeor, 17, 601–613.
Tuller, M., and D. Or, 2001. Hydraulic conductivity of variably saturated porous media: Film and corner flow in angular pore space. Water Resour. Res., Vol.37, No.5, 1257-1276.
Tuller, M., D. Or, and L.M. Dudley, 1999. Adsorption and capillary condensation in porous media -liquid retention and interfacial configurations in angular pores. Water Resour. Res., Vol.35, No.7, 1949-1964.
Ünlü, K., D.R. Nielsen, J.W. Biggar, and F. Morkoc. 1990. Statistical parameters characterizing the spatial variability of selected soil hydraulic properties. Soil Sci. Soc. Am. J. 54:1537-1547.
Ünlü, K., M.L. Kavvas, and D.R. Nielsen. 1989. Stochastic analyses of field measured unsaturated hydraulic conductivity. Water Resour. Res. 25:2511-2519.
Venkatraman, A., Boateng, A.A., Fan, L.T., Walawender, W.P. 1996. Surface fractality of wood charcoals through small-angle X-ray scattering. Aiche Journal 42(7), 2014-2024.
Vereecken, H., T. Kamai, T. Harter, R. Kasteel, J. Hopmans, and J. Vanderborght. 2007. Explaining soil moisture variability as a function of mean soil moisture: A stochastic unsaturated flow perspective. Geophys. Res. Lett. 30:L22402, doi:10.1029/2007GL031813.
Vereechen, H., A. Schnepf, and J. Hopmans, et al., 2016. Modeling soil processes: Review, key challenges and new perspectives, Vadose Zone Jour., 15, 10.2136/vzj2015.09.0131
Vogel, H.J. 2000. A numerical experiment on pore size, pore connectivity, water retention, permeability, and solute transport using network models. Eur. J. Soil. Sci. 51:99-105.
Vogel, H.J., J. Tolke, V. P. Schultz, M. Krafczyk, and K. Roth, 2005. Comparison of a lattice-Boltzmann model, full morphology model, and a pore network model for determining capillary pressure-saturation relationships. Vadose Zone J. 4, 380-388.
Volk, E., Iden, S. C., Furman, A., Durner, W., & Rosenzweig, R., 2016. Biofilm effect on soil hydraulic properties: Experimental investigation using soil‐grown real biofilm. Water Resour. Res, 52(8), 5813-5828.
Wang, X.P., M.H. Young, Z. Yu, X.R. Li, and Z.S. Zhang. 2007. Long-term effects of restoration on soil hydraulic properties in revegetation-stabilized desert ecosystems. Geophys. Res. Lett. 34.
Wardle, D.A., L.R. Walker, and R.D. Bardgett. 2004. Ecosystem properties and forest decline in contrasting long-term chronosequences. Science. 305:509-513.
Wendroth, O., S. Koszinski, and E. Pena-Yewtukhiv. 2006. Spatial association between soil hydraulic properties, soil texture and geoelectrical resistivity. Vadose Zone J. 5:341-355.
Wendroth, O. O.B., Lascano, R., Ma, L., 2018. Bridging among disciplines by synthesizing soil and plant processes, Advances in Agricultural Systems Modeling Vol. 8, Madison, WI, 269p.
Wendroth, O., S. Nambuthiri, and R.J. Walton. 2013. Accounting for soil spatial variability in soil water capacitance probe calibration. Vadose Zone J. 12: 2: -doi:10.2136/vzj2012.0182.
Wendroth, O., W. Pohl, S. Koszinski, H. Rogasik, C.J. Ritsema, and D.R. Nielsen. 1999. Spatio-temporal patterns and covariance structures of soil water status in two Northeast-German field sites. J. Hydrol. 215:38-58.
Wendroth, O., V. Vasquez, and C.J. Matocha. 2011. Field experimental approach to bromide leaching as affected by scale-specific rainfall characteristics. Water Resour. Res. 47, W00L03, doi: 10.1029/2011WR010650.
Western, A.W., S.L. Zhou, R.B. Grayson, T.A. McMahon, G. Blöschl, and D.J. Wilson. 2004. Spatial correlation of soil moisture in small catchments and its relationship to dominant spatial hydrological processes. J. Hydrol. 286:113-134.
Wildenschild, D., J.W. Hopmans, C.M.P Vaz, M.L. Rivers, D. Rikard, and B.S.B. Christensen. 2002. Using X-ray computed tomography in hydrology: Systems, resolutions, and limitations. J. Hydrol. 267:285-297.
Williams-Nguyen, J., Sallach, J.B., Bartelt-Hunt, S., Boxall, A.B., Durso, L.M., McLain, J.E., Singer, R.S., Snow, D.D., Zilles, J.L., 2016. Antibiotics and antibiotic resistance in agroecosystems: State of the science. Journal of Environmental Quality 45(2), 394-406.
Wraith, J.M., and J.M. Baker. 1991. High-resolution measurement of root water uptake using automated time-domain reflectometry. Soil Sci. Soc. Am. J. 55:928-932.
Xing, Y-N., Chen, X-J., Chen, X., and Zhuang, J., 2016. Colloid-mediated transport of pharmaceutical and personal care products through porous media. Scientific Reports 6, 35407.
Yang, Y., and O. Wendroth. 2014. State-space approach to analyze field-scale bromide leaching. Geoderma 217-218:161-172.
Yang, Y., O. Wendroth, and R.J. Walton. 2013. Field-scale bromide leaching as affected by land use and rain characteristics. Soil Sci. Soc. Am. J. doi:10.2136/sssaj2013.01.0018.
Yeh, T. C., L. W. Gelhar and A. L. Gutjahr. 1985. Stochastic analysis of unsaturated flow in heterogeneous soils, 1. Statistically isotropic media. Water Resour. Res., 21(4):447-456.
Zalewski, M., G.A. Janauer, and G. Jolankai. 1997. Ecohydrology: A New Paradigm for the Sustainable Use of Aquatic Resources. International Hydrological Programme, Paris.
Zeiler, M.D. and R. Fergus, 2014. Visualizing and understanding convolutional networks, Lect. Notes Comput. Sci. (including Subser. Lect. Notes Artif. Intell. Lect. Notes Bioinformatics), vol. 8689 LNCS, no. PART 1, pp. 818–833.
Zhang, X., L. K. Deeks, A. G. Bengough, J. W. Crawford, and I. M. Young. 2005. Determination of the soil hydraulic conductivity with the lattice Boltzmann method and soil thin-section technique. J. Hydrol. 306, 59–70.
Zheng, W., X. Yu, and Y. Jin. 2015. Considering surface roughness effects in a triangular pore space model for unsaturated hydraulic conductivity. Vadose Zone J. doi:10.2136/vzj2014.09.0121, 14(7).
Zheng, W., Zeng, S., Bais, H., LaManna, J. M., Hussey, D. S., Jacobson, D. L., & Jin, Y. 2018. Plant growth‐promoting rhizobacteria (PGPR) reduce evaporation and increase soil water retention. Water Resour. Res. 54(5):3673-3687.
Zhu, J., B.P. Mohanty, and N.N. 2006. On the effective averaging schemes of hydraulic properties at the landscape scale. Vadose Zone J. v.5. pp.308-316.