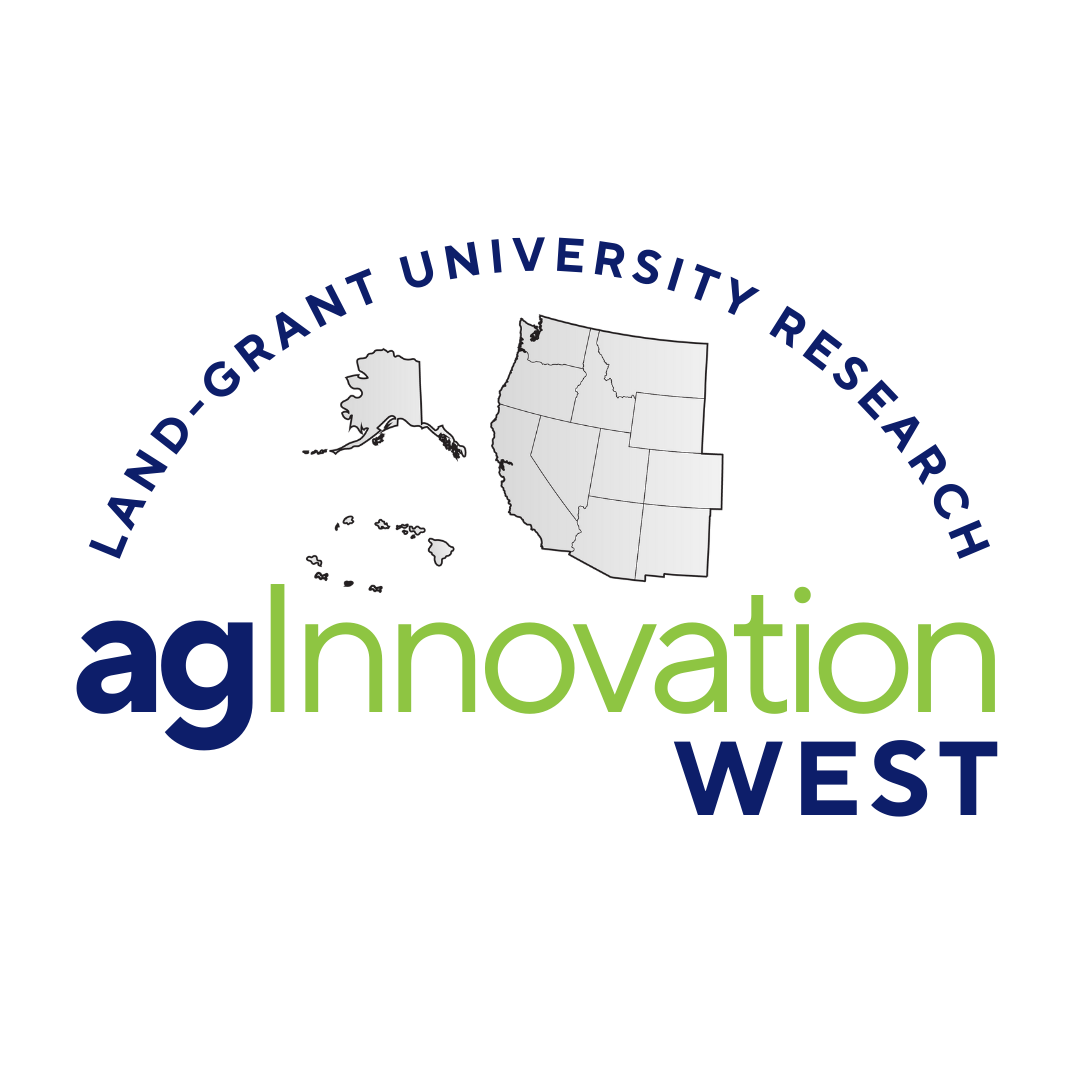
W4128: Microirrigation: A Sustainable Technology for Crop Intensification and Improved Crop Productivity
(Multistate Research Project)
Status: Inactive/Terminating
W4128: Microirrigation: A Sustainable Technology for Crop Intensification and Improved Crop Productivity
Duration: 10/01/2019 to 09/30/2024
Administrative Advisor(s):
NIFA Reps:
Non-Technical Summary
Statement of Issues and Justification
Intensified but sustainable crop production will be a key factor in addressing one of the greatest challenges of this century: feeding 9.5 billion people by the year 2050. Inherent in this challenge are the limitations of arable land as well as a shortage of fresh water sources. Ecologically, crop intensification can protect marginal lands from further development and save water resources. Intensification on a smaller land area also has potential to reduce crop production inputs and crop protection inputs. These include seed, fertilizer, herbicides, pesticides, crop scouting, crop insurance, harvesting costs and any other input cost that has a fixed cost per land area basis. Irrigated agriculture provides about 40% of the world’s food supply utilizing approximately 25% of the land resource. Although there was a great expansion of irrigated lands in the 20th century, most experts agree that such additional great expansion in the 21st century will not be possible. The agricultural community must not only increase the food supply, but it must also conserve water and protect water quality. Crop water productivity (CWP, also known as water use efficiency, WUE) is defined as the crop yield divided by the total water used to produce that crop. Thus, it can be easily recognized that either the numerator can be increased, or the denominator can be decreased to increase CWP. Often strategies to increase CWP concentrate on the denominator such as using deficit irrigation to reduce water withdrawals. Implicit with these strategies is the desire to not greatly reduce farm profitability by negatively impacting crop yields to a large extent. There are limitations to using the denominator to increase CWP, since the overall reason irrigation is practiced is to increase farm profitability. It can also be shown that appropriate levels of irrigation can increase CWP. In that aspect, microirrigation (MI) has great potential to intensify crop production at a greater level while still efficiently using water and also protect water quality.
Although MI is widely recognized as the most efficient and environmentally friendly method of irrigation, the US and international land area is still relatively low. Slowly, this is changing as water resources are stretched and competition for those supplies increases, more synergistic combinations of crop production technologies are developed, and the sheer need for food production increases. Microirrigation will play a large role in these highly-productive agricultural systems. Efforts such as described in this project W4128 add to our abilities to intensify crop production with microirrigation resulting in more sustainable systems that have less negative impact on the environment.
Unless timely action is taken, it is anticipated that water supply- and water quality related crises will affect economies and resources of national and global importance. Microirrigation can reduce the waste of water to a negligible amount and reduce the transport of contaminants to surface water and groundwater. Irrigation events can be fine-tuned to spoon feed water and nutrients just in time to minimize plant water stress. It can optimize crop production (more crop per drop) and in many cases increase the quality of agricultural products.
Related, Current and Previous Work
Results of CRIS Search and related NIFA multistate research projects
A recent CRIS search (January 2019) indicated 999 records related to microirrigation (111), micro-irrigation (258), micro irrigation (258), drip irrigation (820) or trickle irrigation (45) although the ASABE has defined the preferred spelling as microirrigation (ASABE Soil and Water Division Terminology, ASAE S526.3). There were 137 active records which would also include current W3128 activities. Most of these active records can be categorized into 4 major categories that are not well related to the goals of the proposed W4128. These categories include a large number of projects that are primarily using microirrigation as a highly uniform irrigation or water application method to minimize experimental variability, a moderate number of studies where microirrigation (MI) is desirable for minimizing pest and disease control within the research, a moderate number of projects trying to extend using microirrigation to indigenous peoples or to traditionally underserved communities, and a lesser but growing number of projects related to using microirrigation in urban agriculture. The search found 19 records that could be classified as at least somewhat related to the efforts of the proposed project. Ten of these 19 records were either the current umbrella projects of the projected W3128 participants or were being conducted by colleagues at the same location. None of the related projects revealed in the CRIS search appeared to show any unnecessary duplication or overlap.
Review of Progress in Current W3128 Microirrigation Project
This multistate project has been working since its initiation in 1972 to address practical issues related to application of microirrigation technology. Originally formulated as a western U.S. regional project concerning drip and trickle irrigation (W128), over the years the group has included participants from multiple disciplines, such as agricultural engineers, plant and soil scientists, and agricultural economists from diverse regions (Caribbean to the Pacific U.S. Territories). The project was renumbered W1128 in 2004, W2128 in 2009 and became W3128 in 2014. A major project accomplishment during the early years of the microirrigation project W128 was the publication of the original reference book, “Trickle Irrigation for Crop Production” (Nakayama and Bucks, Eds., 1986). The author group of that books successor, “Microirrigation for Crop Production” (Lamm, Ayars, and Nakayama, Eds., 2007) involved 18 past and present project members. In a plenary paper at the 5th International Microirrigation Congress, Phene (1995) attributed the bulk of coordinated microirrigation research to the regional projects W128 and the now defunct S-247. A significant product of the earlier W2128 was the comprehensive microirrigation maintenance website http://micromaintain.ucanr.edu/ with joint collaboration of CA, TX, and KS. The project has offered project-related technical sessions at the Irrigation Associations (IA) conferences in 2001, 2002, 2004, 2005, 2009, 2012, and 2018. Most of these proceedings papers can be accessed at http://www.irrigation.org/technicalpapers. The project has a long history of productivity through publications with 139 publications occurring under the previous project number, W3128 during the period 2014 through 2018. The W4128 project maintains its own website, http://www.cropinfo.net/MI/, and brands some of its creations with the adopted microirrigation logo. The project W3128, “Scaling Microirrigation Technologies to Address the Global Water Challenge”, had three objectives: 1) Develop robust and appropriately-scaled methods of irrigation scheduling using one or more soil-, plant- or weather-based approaches; 2) Develop microirrigation designs and management practices that can be appropriately scaled to site-specific characteristics and end-user capabilities; and 3) Develop technology transfer products for a diversity of stakeholders to promote adoption of microirrigation.
Overall accomplishments of the current project have been excellent as evidenced by the publications from 2015 to 2018. The project participants are agricultural engineers, plant scientists and soil scientists which has been a healthy, synergistic mix of expertise. The participants remain committed to technology transfer as evidenced by including it as a distinct objective in both W3128 and the proposed W4128.
The accomplishments of the current project W3128 directly feed into further advances in microirrigation technologies that are envisioned in W4128 that can increase sustainability and improve water productivity. In some cases the proposed W4128 effort will advance the technology (e.g., combining of irrigation scheduling approaches to increase robustness) while in other cases the effort will concentrate on packaging and promoting the technologies (e.g., smart phone applications of decision tools) that have already been developed in the history of this project since 1972.
Current and Related Research Specific to Obj. 1
Summaries of comparison of irrigation systems, although often being a research topic, are often fragmented covering only certain aspects such as one or a few crops, spatial or geographical aspects such as field shape or slopes, soil or climatic applicability issues, and of course economics. Detailed, comprehensive studies are often limited because of the difficulty in statistically replicating irrigation systems in field studies. Kisekka et al. (2017) compared mobile drip irrigation (MDI) to low elevation spray application (LESA) with center pivots in western Kansas and found evaporative losses could be reduced as much as 35%. They also reported less wheel rutting which can be a late season problem with center pivot irrigation. Other studies of MDI comparisons to alternative systems are currently underway in the Great Plains region, but have not yet reached publication stage. In a review of usage of subsurface drip irrigation (SDI) for tomato, cotton, corn and onion production, Lamm (2016) concluded that there were different reasons for selection of SDI over alternative systems. Cotton and tomato yield increases of 19% and 12%, respectively, with SDI as compared to alternative systems seemed to be an overriding reason in SDI land area increases for cotton in Texas and the southwest and for tomato in California. Additionally, federal cost-share programs implemented in Texas had a large influence on SDI adoption. When averaged over all the reported system comparisons for corn, Lamm (2016) reported only about a 4% yield increase with SDI over alternative systems (e.g., center pivot sprinklers or furrow irrigation) . It was concluded economic competitiveness for SDI as compared to center-pivot sprinklers arises from SDI being able to irrigate a greater fraction of the field area and is improved when corn selling prices and grain yields are higher. For onion, the primary reason for adoption of shallow SDI systems over alternative systems was in the greater number of large and jumbo sized onions which can obtain a market premium. These results indicate there are opportunities to increase MI system adoption and thus increase agricultural sustainability and improve water productivity. However, the reasons for adoption can be diverse. Obviously, economics remains a large impediment to adoption of MI systems, but that continues to change. Key factors affecting MI adoption have been discussed by Wichelns (2007) and Lamm et al. (2015).
Universities in TX and KS and the USDA-ARS sites in TX (which are also participants in this proposed project) have considerable joint research activity concerning development of SDI as part of the USDA-ARS Ogallala Aquifer Program. KS has maintained a website devoted to SDI topics (SDI in the Great Plains, http://www.ksre.ksu.edu/sdi/) since 1998. Although there have been some very good summaries and discussion of SDI research efforts (Camp, 1998; Camp et al., 2000; Ayars, et al., 1999; Lamm and Trooien, 2003; Lamm and Camp, 2007) which have outlined many of the design considerations for SDI from a conceptual standpoint, there still remains a need to develop management procedures and design adaptations as SDI expands into new areas with different climates, soils and cropping systems. The proposed project can help to develop new and broaden existing guidelines, and to package the recommendations into more easily accessible platforms.
When considering nutrient or agrochemical application in conjunction with microirrigation, water quality protection must be considered in addition to improving crop production and improving agrochemical efficacy. Groundwater quality can greatly affect the sustainability of agriculture production because salinity produces an important abiotic stress and exacerbates the plant water stress further (Miyamoto and Storey, 1995; Deb et al., 2013a). Salinity stress is an ever-present environmental constraint to crop productivity in arid and semiarid regions and thus can affect the scaling and selection of irrigation systems. Salts present in the soil and irrigation water are expected to increase as the competition for freshwater uses among domestic, agriculture, and industrial sectors intensifies.
Among all the elements needed for plant growth, nitrogen (N) is considered the most important fertilizer element applied to soils because crop requirements for N are high compared with requirements for phosphorous (P), potassium (K), and other essential plant nutrients (Allison, 1996). However, solubility of nitrate (NO3) sources in water can cause rapid movement through soils, and among the various pathways of N loss in agricultural fields, leaching is considered a major source of NO3-N loss under normal agricultural practices (Allison, 1996). Crops differ in rooting depths, rooting densities, N and water requirements, and plant uptake efficiencies (Peterson and Power, 1991), and the percolation of NO3-N to deeper soil layers depends on the cropping systems. In addition to N fertilizers and water applied by irrigation or received through precipitation, type of irrigation system and soil physical properties also play important roles in NO3-N leaching to groundwater (Al-Jamal et al., 1997; Cepuder and Shukla, 2002). In arid regions excess irrigation is also applied to flush salts out of the rooting zone to control soil salinization, potentially leading to high N leaching. Microirrigation can supply water and N directly to the crop root zone and can potentially reduce water and NO3-N leaching to the deeper soil layers. Drip irrigation systems have been reported to apply 22% (Bucks et al., 1974) to 30% (Gustafson, 1975) less water than furrow irrigation systems. Higher onion yields, larger bulb sizes, less NO3-N leaching, higher water use efficiency, and higher N fertilizer use efficiency were reported under drip irrigation systems compared to furrow irrigation systems (Halvorson et al., 2008).
The use of sensors, irrigation scheduling and other technologies and practices to improve management of MI and alternative systems and irrigation scheduling is an emerging research topic in many locations around the US. Technologies that can help to improve or optimize irrigation management include automated weather stations, unmanned aerial vehicle (UAVs) or satellite-based remote sensing platforms, automated monitoring and control; systems, plant water stress sensors, and soil water sensors (Broner 2005; Martin , 2009) Interest in soil water sensors, particularly those that can be interrogated remotely is growing and information they provide have been shown to be effective and to improve irrigation management (Martin et al., 1995). These sensors when used in irrigation scheduling have been shown to increase crop yields while conserving water (Martin et al., 1995; Fisher et al., 2009; Sui, 2017, Kebede et al., 2014). In Florida, Zotarelli et al. (2009) reported that users who managed irrigation with soil water sensors applied less irrigation water (15% to 51%) compared to typical fixed-time irrigation plans and observed yield increases of 11% to 26%. In addition, modern sensors often provide continuous readings without crop interference and are usually cost effective, requiring little or no maintenance over their anticipated lifetime (Cardenas-Lailhacar and Dukes, 2010), although above-ground telemetry and other components can be vulnerable to damage. There is a wide selection of soil moisture sensors to choose from including tensiometers, neutron gauges, electromagnetic sensors, electrical resistance sensors, and heat dissipation sensors among others (Yoder et al., 1998).
Additional discussion of sensors and irrigation scheduling are discussed under Objective 2.
Current and Related Research Specific to Obj. 2
Irrigation scheduling and water management take on many forms (Hoffman et al., 1990). Some of these techniques are soil-based, some are plant-based, some are weather-based and some are a combination of two or more approaches.
Much of the current research emphasis on irrigation scheduling has focused on models of crop water demand, based on one or more weather-related factors that describe the evaporative demand of the atmosphere (e.g., FAO 56 in Allen et al., 1998). This approach assumes that the water use of any particular crop (the crops evapotranspiration, or ETc) can be calculated as a simple proportion of the calculated reference ET from a reference surface (typically either a short grass surface or a taller alfalfa surface) under the same weather conditions, using the relation ETc = Kc x ETref. The empirical proportionality constant (crop coefficient, Kc), is meant to include all effects specific to the crop in question (stage of canopy and plant development, crop health, degree of water stress, etc.), and many different resources have been developed to help growers adapt this approach for irrigation scheduling (Gowda et al., 2011, Rogers, 2012). This approach is very useful for planning purposes at a regional scale, but it requires a number of assumptions and/or site specific information (e.g., local rainfall, crop rooting depth) in order to be scaled appropriately for on-farm use. It also requires environmental information appropriate to the microclimate that is experienced by the crop, and in some cases this information is not widely available, or only available at a regional scale. One of the significant issues with the FAO Kc x ETref approach is the non-transferable nature of the Kc coefficients from one location to another, which justifies the need to develop alternative and more robust methodology for estimating crop water requirements. Hence, there is a need for continued development of this approach, in order to supply growers with more accurate and site-specific (e.g., growing degree day-based) or microirrigation system-specific (e.g., DI vs. SDI) estimates of Kc, forecast ET (reference) information for on-farm planning purposes, and web-based access to models that either measure or calculate local weather conditions (e.g., rainfall). Such improvements will be useful to growers as well as water planning authorities (e.g., Fares, 2013).
Soil-based approaches to irrigation scheduling (e.g., Casanova et al., 2012) are an alternative that may be more directly scalable to the needs of the grower than weather-based approaches, since they are based on local soil water measurements. Since both soil- and weather-based approaches are founded on the same principles of crop water balance, they should give comparable results, and hence combining these approaches may provide mutual support for growers’ irrigation decisions. Soil water content is an important metric for managing timing and amounts of irrigation (Merriam, 1966; Evett et al., 2009). Due to the large rooting depth of some crops, measurements of the soil water profile down to >2 m are necessary in some cases to accurately capture the root zone soil water dynamics, particularly for soil water balance studies of crop water use. Passive and active microwave remote sensing are capable of sensing soil water, but only to depths of approximately 5 cm (Jackson and Schmugge, 1989). Neutron probes are accurate and can be used to appropriate depths, but are impractical for frequent measurements (less than a few days), are labor intensive, and come with regulatory burdens due to the radiation source. Capacitance probes are often inaccurate due to heterogeneity in soil electrical conductivity and structure (Mazahrih et al., 2008; Evett and Steiner, 1995, Evett et al., 2009). Time-domain reflectometry (TDR) uses the travel time of an electric pulse sent down a waveguide in the medium to be measured (Topp et al., 1980). Travel time is related to the soil complex dielectric permittivity and is affected by pulse bandwidth and soil bulk electrical conductivity, with temperature effects on bound water and conductivity, as well as soil water content effects on conductivity. For TDR, these complicated interferences can be accurately calibrated by accounting for bound water and temperature effects (Schwartz et al., 2009). However, these calibrations are tedious, and conventional TDR probes are not easily installable to the depths required, so additional work in this area is needed.
Since the final objective of irrigation is to economically improve plant productivity, plant-based irrigation scheduling should in theory be scale independent, but plant responses to water limited conditions are complex (Jones, 2004), and the availability of reliable and relatively low-cost approaches for grower use are currently limited. Infrared thermometers (IRTs) have been widely used in agricultural research to remotely measure surface temperatures and develop vegetation stress indexes (Jackson et al., 1981; Colaizzi et al., 2003), monitor crop canopy temperatures and schedule irrigations based on plant feedback (Jones, 2004; Peters and Evett, 2007), predict crop yields (Irmak et al., 2000; Ajayi and Olufayo, 2004), or estimate sensible and latent heat fluxes (e.g. Kustas and Norman, 1997; Kalma and Jupp, 1990). Historically, these applications employed the use of stationary industrial IRTs wired to data loggers. These sensors can be expensive, cumbersome, and impractical for commercial on-farm applications. Recent wireless technology (O’Shaughnessy and Evett, 2010a; O’Shaughnessy et al., 2011) and internet access have improved the prospects for on-farm use of these devices, but further research is needed to evaluate these devices and to develop user-friendly systems and scheduling criteria that are appropriate to the crop of interest and the device used. The most direct, but also labor and/or technology intensive plant-based irrigation scheduling method is the measurement of plant water status, typically as measured by plant water potential. While the relevance of this measure to plant function has been questioned (Sinclair and Ludlow, 1985) a few early studies in woody perennial crop species (Garnier and Berger, 1985, McCutchan and Shackel, 1992) reported a close relation of mid-day stem water potential to both irrigation regime and plant physiological activity. This approach has been successfully used for deficit irrigation strategies in high value woody perennial crops (Shackel, 2011), but its application to herbaceous crops, while successful, has been rare (Campillo et al, 2008). The applicability of this approach to a wide range of crop species is warranted, as it can either serve as direct feedback to irrigation scheduling (e.g., Girona et al, 2006), or as a research tool to evaluate other indirect plant-based measures that can be automated, such as canopy temperature (Peters and Evett, 2008; O’Shaughnessy and Evett, 2010b) or stem diameter fluctuations (Conejero et al, 2011).
Many of the participants have a long history of being involved in irrigation scheduling and jointly their expertise and experience will be applied to advance microirrigation scheduling methods that can increase sustainability of water resources and improve water productivity. The advances in sensors and communication technologies that are anticipated for the agriculture sector in the next 5 years will be advantageous for highly efficient MI systems.
Current and Related Research Specific to Obj. 3
Currently, there are many efforts underway in the US to use autonomous or nearly autonomous software programs to manage irrigation for MI and alternative irrigation systems. Current participants of the W4128 project are involved in many of these efforts, which involves intellectual property valuable to the developers and hence have not been fully discussed in publications. Most notably in the context of this proposal are efforts underway by W4128 participants in CA, TX, and USDA-ARS. Recently, Evett et al. (2018) from USDA-ARS (also a W4128 participant) discussed how cloud computing could address field water monitoring and improve irrigation management. In another recent publication, Andrade et al. (2018) discussed how machine learning could be used to forecast crop water stress. Research being conducted by Kisekka (2019) and colleagues at UC-Davis (also a W4128 participant) will couple the DSSAT model with soil and weather databases that will be accessed automatically to both model crop production and schedule irrigation. The effort is being called iCrop.
For almost 90 years, it has been recognized that the movement of water and energy in the soil are coupled (Boucoyous, 1915). However, soil water movement in unsaturated soils is mostly studied under isothermal conditions based on the Richards equation (Richards, 1931). Early studies (Taylor and Cavazza, 1954) demonstrated that moisture movement in response to thermal gradients through an unsaturated soil occurs mainly in the vapor phase. The complexity of the coupled liquid water, water vapor and heat transport in the unsaturated zone and the difficulties associated with field measurements especially near the soil surface necessitate the application of numerical models to analyze these processes (Deb et al., 2011). Water transport under both liquid and vapor phases were studied involving laboratory (Hanks et al., 1967; Westcot and Wierenga, 1974; Nassar and Horton, 1992) and field (Ross et al., 1985; Scanlon and Milly, 1994; Saito et al., 2006; Deb et al., 2013a) conditions. There have been limited modeling studies that evaluated coupled fluxes and their temporal variations in field soils where irrigations are commonly applied after short intervals (Deb et al., 2013b). The growing computational capacity has enhanced the use of more accurate simulation models coupled for water and energy transport in unsaturated soils (Saito et al., 2006). Solute transport in and out of the root zone can be simulated using a variety of numerical models. Among these models are the Nitrate Leaching and Economic Analysis Package (NLEAP; Shaffer et al., 1991), Agriculture Production Systems Simulator (APSIM; McCown et al., 1996), Root Zone Water Quality Model (Ahuja et al., 2000). These models can also provide deeper insight into the transport behavior as well as leaching of applied chemicals and fertilizers toward the groundwater table with irrigation. Several successful applications of the HYDRUS model are also available in the literature (e.g., Kandelous and Šimůnek, 2010; Deb et al., 2011). HYDRUS has been applied in diverse scenarios of different land uses and management systems, as well as for different irrigation and fertigation practices (e.g., Hanson et al., 2006; Ebrahimian et al., 2013; Deb et al., 2015; Šimůnek, et al., 2016; Levy et al., 2017; Šimůnek, et al, 2018). A recent review of literature identified at least 90 manuscripts in which HYDRUS had been used to simulate microirrigation systems (Šimůnek, 2019).
The presence within the proposed W4128 project of these important research teams and their extensive knowledge and experiences with modeling will be very beneficial in advancing MI systems and technologies that can lead to greater sustainability and improved water productivity.
Current and Related Research Specific to Obj. 4
As this Objective relates directly to technology transfer rather than a specific research topic, the discussion for technology transfer was discussed in the earlier Section “Review of Progress in Current W3128 Microirrigation Project”. Participants on the project include applied research and extension faculty with extensive experience and established outreach infrastructure. It is anticipated that the long history of extensive and concentrated technology transfer will be continued in W4128.
Objectives
-
Develop and evaluate irrigation systems, designs, technologies, and management practices that are sustainable and can increase water productivity.
-
Improve methods of irrigation scheduling that are particularly applicable for microirrigation.
-
Develop, evaluate, and adapt models as tools to improve system design, management, and production.
-
Expand technology transfer products for a diversity of stakeholders to promote adoption of water-saving irrigation strategies.
Methods
Methods for Objective 1 (Designs, Technologies and Management Practices)
Irrigation system selection, designs, technologies, and management practices are key factors contributing to sustainable use of water resources and increased water productivity. Nearly all participants will make contributions to this Objective, but direct participants will include AL, AZ, CA-UCD, CA-UCR, FL, ID, KS, NE, NM, NY, OK, OR, TN, TX-TAMU, USDA-ARS, WA. This broad group of participants provides a diversity in crop, soil, climate, water availability, and governmental and other constraints. This diversity can foster a more complete and robust conceptual addressing of the 4 key factors in Objective 1.
Sub-Objective 1a (Improved System Designs and Irrigation System Comparisons)
Alternative irrigation systems are often selected instead of microirrigation systems (MI), although the latter can often make more efficient use of water resources. Center pivot sprinkler irrigation systems (CP) are the predominant irrigation system in the United States for grain, fiber, and oil seed crops and will continue to be so for quite a while into the future. Mobile drip irrigation (MDI), where driplines are being attached to CP systems, may be a means to further extend MI technologies into a larger proportion of the US irrigated area. Subsurface drip irrigation (SDI) although more expensive than CP or CP systems with MDI also is being adopted by some farmers. ID, KS, and USDA-ARS will evaluate and further develop MDI technologies for irrigating crops, such as maize and cotton, in their individual region. In some cases, MDI will be compared to other types of CP application packages while in others it will be compared to SDI. Joint efforts to look at defining appropriate slope steepness and soil textures to avoid excess runoff for CP and MDI are planned. KS, TX-TAMU, and USDA-ARS will compare SDI and CP systems for crop production in terms of yield, water and energy use, water losses such as evaporation and runoff, crop water productivity, and the associated economics for appropriate crops in their region. The combination of data sets from these efforts will provide for a better understanding of the MI and CP selection process, as well as identifying future research to reduce barriers to the more efficient MI systems.
The need for development of cost-effective, profitable SDI systems in the US Great Plains, the Southwest, the Pacific Northwest and the Southeast, as well as internationally, remains an important research topic. AZ, CA, KS, NE, OK, TX-TAMU, USDA-ARS, and WA will continue strong research programs in this area. AZ, CA-UCD, KS, and USDA-ARS will examine alfalfa production with SDI with AZ using Hydrus II-D to examine soil mechanics and soil physics aspects, including soil trafficability, while the other partners are focusing on both crop production and design issues that can increase water productivity. KS, NE, and USDA-ARS in cooperation with international partners, will continue efforts to scale and adapt SDI and other MI technologies for use by low-input producers that can be either domestic or international. KS, NE, OK, TX-TAMU, and USDA-ARS will evaluate SDI designs (depths, spacings, orientations) under different irrigation and fertilization regimes in terms of production, water use, water productivity and economics for crops grown in the semi-arid Great Plains region. Effective procedures and techniques for fertigation will be an SDI research topic for KS, NE, OK, and WA with a focus on improving production, improving nutrient efficacy, reducing environmental hazards, an improving net farm income. In particular, WA will focus research on a newer novel method of applying water and nutrients at deeper depths in the profile applicable for vine crops, while other participants will focus more on grain crops.
Sub-Objective 1b (Sensors and Technologies for Improved Microirrigation Management)
The use of sensors and other technologies to improve management of MI systems and irrigation scheduling, and to develop precision irrigation schemes/methods is a large focus area of the proposed project involving AL, CA, ID, FL, KS, NE, NM, NY, OK, OR, TN, TX, USDA-ARS, WA. Greater detail on the efforts with regards to irrigation scheduling will be discussed under Objective 2. Management schemes, ultimately for precision microirrigation, using sensors for monitoring and control will be developed and evaluated by CA, KS, NE, NM, NY,OK, OR, TX, and USDA-ARS. These schemes will range from simple information delivery for decision support to more fully autonomous systems that can be overridden by the enduser should conditions warrant. As an example CA and NY are working on evaluating electronic tensiometers with an extended range of operation (greater than typical 1 bar). This effort will be discussed more under Objective 2. On the other end of the spectrum, CA, KS, OR, TX, and USDA-ARS will be evaluating more fully autonomous irrigation management systems. As this is a rapidly emerging research topic, it is anticipated that these results will inform future research decisions. Application of UAVs and remote sensing will be focus areas for AL, KS, and USDA-ARS. There are also large technology transfer efforts to disseminate sensor technologies to producers in AL, CA, FL, ID, KS, NE, NM, OK, TN, TX, and WA.
Sub-Objective 1c (Nutrient Management Schemes and Technologies for Microirrigation)
Optimizing crop water productivity under irrigation system requires careful attention to nutrient management. However, MI systems are well suited to applying nutrients both in a spatial (e.g. near plant roots with less off-site movement) and temporal (just-in-time while minimizing excesses or shortages) contexts. Crop water productivity is increased by having the nutrients optimized in both the spatial and temporal sense. Many, if not all of the W4128 participants will utilize precise nutrient management to increase crop yields, improve water productivity, as well as increase sustainability of agriculture systems through minimizing contamination and pollution. Nutrient management technologies, procedures and strategies for higher-value fruit, vegetable, tree and vine crops are often more complex than those of lower-valued grain, fiber and oil seed crops, but many of the technologies can be economically scaled or adapted to the pertinent crop. CA-UCD, CA-UCR, FL, OR, TN, USDA-ARS, WA will focus some irrigation and nutrient management research on these higher value crops while KS, NE, NM, OK, OR, and WA will conduct similar types of studies with lower value or commodity crops. Modeling efforts by CA-UCR, CA-UCD and NM will augment and extend the results from these studies.
Methods for Objective 2 (Irrigation Scheduling)
Irrigation scheduling based on ET, Soil, Plant, or combined methods will be an integral part of the research conducted by essentially all project participants.
Sub-Objective 2a (Weather or ET-Based Irrigation Scheduling)
Weighing lysimeters represent a ‘gold standard’ for measuring ET and determining Kc values, and both CA-UCD and USDA-ARS will continue to operate large weighing lysimeters for this purpose. Both locations will also be used to compare ET approaches with alternative (soil- or plant-based) approaches, and at both locations the impact of irrigation management on crop coefficient values will be determined. ET- or combined ET/soil-based scheduling software will also be developed for agricultural crops and urban landscapes. TX-PVAMU and CA-UCR will both have efforts in developing better scheduling procedures for urban landscapes. TX-PVAMU, for instance has the Irrigation Water Estimator for Texas (IWET) which uses the Irrigation Management System (IManSys) model to estimate crop irrigation requirements for both agricultural crops and urban landscape in Texas. Recently, the tool was updated for multiple users to use the model simultaneously. The major input data used in this tool are site-specific soil data based on the Soil Survey Geographic Database (SSURGO), daily gridded climate data (1979-2014) from the Climate Forecast System Reanalysis (CFSR), and crop-specific water uptake data. The user can select the appropriate planting, harvesting dates, and the irrigation system to be used. Based on all these inputs, IManSys calculates the IWR and the major water budget components for different periods, i.e., seasonal/annual, monthly, bi-weekly and weekly. This information is useful for water allocation, irrigation system design, and irrigation scheduling based on historical climate data. A similar concept will be used to develop irrigation water estimator for other states. Smart irrigation controllers and soil moisture sensors will be evaluated regarding their efficiency, reliability, and ease of use in the context of an ET-based experiment in CA-UCR.
Several of the participants (CA-UCD, FL, KS, OK, TX-PVAMU, TX-TAMU, WA, and USDA-ARS) have/shared active weather and ET networks and also have and support weather based irrigation scheduling software.
Sub-Objective 2b (Soil-Based Irrigation Scheduling and Sensor Evaluation)
ID, KS, NM, OK, TX-TAMU, TX-PVAMU, WA, and USDA-ARS will have a strong focus on soil-based approaches as well as the evaluation, calibration, and/or development of sensors and electronic logging systems. Crops studied will include legume and grain cover crops, row crops (leafy greens, okra, tomato, and strawberry), cereals, tree crops, wine grapes, and halophytes irrigated with brackish water. Tests will include calibration of soil moisture sensors in soil amended with different amendment types and rates, and comparison of soil sensor accuracy for soil moisture, soil temperature and soil salinity. Commercial soil moisture sensors will also be used as part of a recently developed prototype automated irrigation scheduling package. Experiments will be performed on experiment station lands and in grower cooperator fields, and all information will be extended to the respective industry clientele, including grower feedback regarding level of satisfaction, convenience and perceived value of the sensors an approaches tested.
Sub-Objective 2c (Plant-Based Irrigation Scheduling and Combined Methods)
CA-UCD and NY will cooperate with industry (FloraPulse, Inc.) to test novel plant-based sensors (microtensiometers) for the direct measurement of plant stress (Stem Water Potential, SWP) for irrigation management in tree and vine crops in commercial orchards/vineyards and experiment station plots. It should be noted that although direct efforts with plant-based irrigation scheduling did not surface appreciably in the revision of this project, information from research studies in Objective 1 and efforts to develop autonomous irrigation control systems in Objective 1 and 3 definitely have plant-based information as integral parts, or at least are feeding information that can be used for validation of technology effectiveness or system operation. There is a continuing trend in system automation to allow weather, soil or plant information to be accessible to end users, and/or to help in directly or indirectly in irrigation management.
Methods for Objective 3 (Modeling and Algorithms for Evaluation and/or Control)
Conclusions from field research can be affected by many uncontrollable factors and more robust conclusions can often be augmented by modeling. In the proposed project, participants from AZ, CA-UCD, CA-UCR, KS, NM, OR, TX-PVAMU, TX-TAMU and USDA-ARS will use models and algorithms to improve design, management, and crop production with MI systems. The goals of these efforts are to improve natural resource effectiveness, environmental quality, crop production, performance and operation of irrigation systems and net farm income.
Sub-Objective 3a (Autonomous/Nearly Autonomous Control and/or Management Systems)
Nearly autonomous irrigation systems operation and management are the goal of modeling efforts of TX-TAMU and USDA-ARS. In these efforts, participants (TX-TAMU and USDA-ARS) will work directly with industry partners to develop and adapt algorithms suitable for incorporation and/or communication with industry hardware. Evaluation and adaptation of industry-developed hardware and software for irrigation management will be the focus of research in KS and OR. The product in this effort is near the marketing stage, but it seem likely that further adaptations to other locations and crop will be warranted. CA-UCD is working on a comprehensive model-driven decision support system, suitable for cloud computing for irrigated crop production called iCrop. Currently, iCrop is setup as a GIS-enabled web application to run with the DSSAT crop model and automatically access soil (e.g., USDA NRCS SSURGO) and weather (e.g., CIMIS) databases.
Sub-Objective 3b (Modeling to Evaluate Microirrigation Strategies)
AZ, NM, and CA-UCR will engage in significant efforts with the suite of Hydrus models to develop improved irrigation, nutrient and salinity management techniques. AZ plans to use Hydrus 2D to evaluate soil mechanics and soil physics for SDI design characteristics for alfalfa management such as soil trafficability, particularly during irrigation events. This topic was also discussed under Objective 1. NM will use Hydrus models to evaluate water movement, ET, and nitrate movement for crops and locations within the state. CA-UCR (as developer of Hydrus suite of models at UC-Riverside) has numerous activities involved with research groups internationally, as well as with W4128 participants. These research efforts, to name a few, include evaluating the water footprint of various irrigation systems and irrigation strategies, evaluating system designs and operational procedures, evaluating irrigation and chemical application strategies for minimizing environmental hazards, and identifying temporal and spatial risks.
The combined efforts under Objective 3 will be useful in developing more robust management schemes for irrigation systems and for the methods for how they are being operated that are suitable for a wider proportion of the irrigated landscape. Additionally, these efforts can identify barriers to adoption and point to further research needs.
Methods for Objective 4 (Technology Transfer)
Another important focus of the project includes development and adaptation of technology transfer products for a diversity of stakeholders. All project participants will have some involvement in technology transfer, whether directly through cooperative extension or more indirectly through publications and presentations. Efforts will focus on four primary activities: development and expansion of internet-based resources, decision tools and applications; development of print and multimedia content; coordination of educational events; and advancement and promotion of microirrigation through public-private partnerships.
Internet-based delivery of information is the mainstay for modern technology transfer, and well-established websites will continue to serve as primary sources of information for many stakeholders. Although the publications and products of the current W3128 project are thoroughly listed on the project website http://www.cropinfo.net/MI/ many of these documents cannot be easily obtained through active Internet links. In the proposed project, all participants will work to improve free and open access to publications and products for global stakeholders. Since much information remains underutilized, project participants will work together to increase availability of information and visibility in audience-appropriate formats.
Participating programs will leverage existing Internet resources to deliver new and expanded tools and information resources, including adapting existing decision support tools or new applications (apps) for smartphones, tablets and other mobile devices, and providing training for end-users. Irrigation management and scheduling tools and apps are available or under development by participants in CA-UCD, FL, KS, TX-PVAMU, TX-TAMU, USDA-ARS, and WA, and new and expanded tools are planned by these participants and others. A very useful product from a previous project (W2128) was the webpage, “Maintenance of Microirrigation Systems”, hosted at http://micromaintain.ucanr.edu/. W4128 participants will explore updating this resource to reflect new developments in maintenance of microirrigation systems.
Higher level integration of information from multiple sources for use in decision support will be made possible through development of new platforms. CA-UCD, TX-PVAMU, and TX-TAMU plans to integrate multiple data streams and models through an Internet-based or cloud-based software packages. KS will work to develop decision tools for MI, with goals to help generalize and standardize the procedural order of questions and the format of input data to successfully use microirrigation. Long term goals are to develop flowchart-driven (decision tree) decision tools to help potential end users or system providers decide if MI is the right choice and to help lead them through the design process.
A variety of outreach strategies will be used to reach diverse audiences. Traditional extension/technology transfer methods (print and electronic fact sheets; field days; presentations at meetings for agricultural and horticultural producers; training events for county extension agents; Continuing Education Units (CEU) opportunities for irrigation professionals and crop advisors, mass media, technical sessions at conferences) will be complemented with newer/emerging methods (social media, YouTube, Twitter, and others) to reach traditional and emerging audiences. Multi-state/multi-institution (USDA-ARS, TX, KS, OK, OR) products (publications, field days, etc.) will reinforce the collaborative nature of the project, and they will promote consistent messaging of the educational efforts.
Building upon existing conventional libraries of content, all participants will expand subject matter and reach a greater diversity of stakeholders by emphasizing audience appropriate presentation (user-friendly language and formats; appropriate technical level) and greater accessibility.
Educational events remain key to technology transfer. Workshops, field days, technical sessions, webinars, and conferences will be achieved through venue-appropriate and audience-appropriate activities. Stakeholder targeted field days, workshops and conferences will be conducted by the Project Participants. These activities may include events sponsored by industry, commodity groups and trade associations. Some activities will build upon foundations of traditional (often perennial) events; others will require coordinating and marketing of new events (additional venues, audiences). Technical sessions or conferences will be organized and supported by all Project Participants, and may be in conjunction with regional, national and international organizations (e.g., The Irrigation Association, American Society of Agricultural and Biological Engineers, Central Plains Irrigation Association). Where applicable, Continuing Education Units (CEU) or Professional Development Hours (PDH) credits will be offered. The project has had success with these outreach outlets in the past. If funding can be secured through another source, the project proposes to conduct a stand-alone technical conference or symposium to be held in the United States during the latter two years of the project.
Public-private partnerships, including cooperative research and development agreements (CRADA) can be highly effective technology transfer strategies. USDA-ARS will continue with these efforts. KS and OR also have cooperative activities with industry partners. Project participants will initiate more formal advancement and promotional activities with industry as appropriate opportunities arise. Anticipated benefits of these alliances will be greater access to microirrigation information and improved conceptualization and generalization of design, installation and maintenance processes, leading to increased adoption of MI.
Measurement of Progress and Results
Outputs
- • (Under SubObj 1a) Knowledge base for comparisons of alternative irrigation systems
- • (Under SubObj 1a) Improved designs and BMPs for subsurface drip irrigation (SDI)
- • (Under SubObj 1b) Knowledge base and application criteria for microirrigation (MI) sensors and technologies
- • (Under SubObj 1c) Knowledge base for nutrient management schemes for MI
- • (Under Obj 2a) Weather-based microirrigation scheduling procedures and technologies
- • (Under Obj 2b) Soil-based microirrigation scheduling procedures and technologies
- • (Under Obj 2c) Plant-based microirrigation scheduling procedures and combined scheduling procedures
- • (Under Obj 2a to 2c) Software and phone applications for microirrigation scheduling
- • (Under Obj 3a) Nearly autonomous irrigation system control and monitoring systems
- • (Under Obj 3a) Improved modeling and evaluation of soil physics processes, and crop production, irrigation, and nutrient management strategies for MI
- • (Under Obj 4) Expansion of existing libraries of publications (print and/or electronic formats) to reach diverse audiences
- • (Under Obj 4) Educational events for diverse audiences
- • (Under Obj 4) Advancement and promotion of microirrigation through public-private partnerships
Outcomes or Projected Impacts
- Comparisons of alternative irrigation systems will allow growers to make the best system choice for their operations and will allow them to optimize performance of existing systems
- Improved design and management procedures for SDI will allow broader penetration of microirrigation into regions of the country where adoption has been limited
- Conceptualization and generalization of microirrigation design and management procedures and tools will allow for growers, technical service providers, dealers, and industry to communicate more easily the requirements and preferences for new system installations
- Soil-, weather-, and plant-based or combined microirrigation scheduling approaches will provide valuable choices for a diverse clientele of growers to improve their crop production and profitability while reducing irrigation withdrawals
- Increased adoption and proficient management of microirrigation scheduling will improve water productivity and promote improved water quality over a wider range of end-user characteristics and constraints
- Nearly autonomous irrigation systems will reduce the day-to-day time management requirements of irrigators and will provide better access to science-based irrigation management
- Risks of negative impacts to environment, soil, and water quality will be minimized through reduced leaching or other off-site /non-target chemical movement possibly opening up previously non-productive lands to microirrigation
- Modeling of soil physics, crop production, irrigation, and nutrient management related to microirrigation will provide for faster and less expensive evaluation of diverse strategies
- Expansion of existing websites and accessibility of publications will allow for increased regional, national and international adoption of microirrigation technology
- Educational events tailored to the audience abilities and needs will increase adoption and correct usage of microirrigation
- Technical sessions and/or conferences will bring together experts representing different approaches to microirrigation; expand the knowledge base; improve networking of scientists and contribute to a more integrated approach to this field of research and education
- Increased collaboration between public and private entities will help to increase adoption of microirrigation on a much broader scale and will improve the correct implementation of this technology
Milestones
(2020):Field studies to evaluate alternative irrigation systems are initiated(2020):Field studies to evaluate single, multiple, or combined irrigation scheduling techniques begins
(2020):Laboratory and field studies to select, develop, calibrate, adapt, and evaluate soil water and/or plant water stress sensors begin
(2020):Field studies will be initiated to determine optimal MI water and nutrient management for various crops.
(2020):Initial discussions and outlines will be developed for both publications and software being jointly developed or adapted to a new locale
(2020):Tours, field days and educational events will be held at the local and regional level to promote microirrigation
(2020):Annual meeting for state reports
(2021):All laboratory, modeling and field studies initiated in 2020 for Objectives 1 to 3 will continue using any strategy adjustments determined useful from first year results
(2021):Map out any new or adjust any existing website decision trees and identify major information and subject matter gaps
(2021):Test software and smartphone applications at their current stage of development (i.e., stage varies between participants and products) and identify gaps
(2021):Continue educational events at local and regional level and evaluate additional needs of those audiences
(2021):Draft extensive publication for SDI design and management for commodity crops
(2021):Conduct roundtable discussions with industry partners to identify needs and possible areas of cooperation
(2021):Identify potential funding sources for national or international conference on microirrigation
(2021):Annual meeting for state reports
(2022):Overall structures of websites will be completed and easily-accessible and audience-tailored content be increased.
(2022):Initial laboratory, modeling, and field studies will be completed and analysis will be underway.
(2022):Identify and initiate any follow-on laboratory, modeling, and field studies needed for project completion.
(2022):Release extensive publication for SDI design and management for commodity crops.
(2022):Evaluate progress with written publications in terms of accessibility and meeting audience needs and begin effort to fill gaps.
(2022):Continue educational events at local and regional level and offer technical session at national conference.
(2022):Make decision on whether to conduct national or international microirrigation conference and appoint planning team if appropriate.
(2022):Continue joint activities with industry and identify new opportunities
(2022):Annual meeting for state reports
(2023):Finish analysis of all initial laboratory, modeling and field studies that began in 2020 and publish results
(2023):Finish any follow-on laboratory, modeling, and field studies that began in 2022 and begin analysis of results
(2023):Finish all software and smart phone application developments and promote products through appropriate venues
(2023):Finish drafts for any publication gaps identified in 2022.
(2023):Plan and prepare for national or international microirrigation conference if positive decision in 2022.
(2023):Reevaluate collaborations with industry and make adjustments
(2023):Plan for revision of W4128 microirrigation project.
(2023):Annual meeting for state reports.
(2024):Prepare W5128 project revision
(2024):Release any remaining software and smart phone applications to public domain
(2024):Finalize all reports and make presentations at local and regional meetings
(2024):Conduct national or international microirrigation conference if positive decision in 2022
(2024):Summarize project-wide accomplishments and present at national or international meetings
(2024):Finish current collaborations with industry
(2024):Initiate W5128 project
Projected Participation
View Appendix E: ParticipationOutreach Plan
Organization/Governance
The organization and implementation of the project will be in accordance with the Manual for Cooperative Regional Research.
The Regional Technical Committee will consist of representatives from each cooperating Agricultural Experiment Station and federal agency cooperating in this project. The representative(s) will be appointed by their respective Experiment Station or Research Director. The above will constitute the voting membership of the technical committee.
The Regional Technical Committee will be responsible for the planning and execution of the research project. It will be responsible for coordinating research activities of each cooperating Experiment Station and federal agency and for the developing of appropriate research methods and procedures.
A Director from the Agricultural Experiment Stations of the Western Region appointed by the Agricultural Experiment Station Directors of the Western Region will serve as Administrative Advisor and an ex-officio (non-voting) member of the technical committee. A representative of the USDA National Institute of Food and Agriculture (NIFA) will serve as an ex-officio (non-voting) member of the technical committee.
An executive committee, consisting of a chair, vice-chair, and secretary will be elected from the voting members of the technical committee. The executive committee will serve one year in each elected office with the provision that the vice-chair will ascend to chair, and the secretary to vice-chair. A secretary will be elected each year. The executive committee will have the authority to act on behalf of the technical committee.
The chair, with the approval of the Administrative Advisor, will notify technical committee members of the time and place of meetings, prepare the agenda, and preside at meetings of the technical committee and executive committee. The chair will also be responsible for naming appointments to subcommittees for specific assignments. The chair will be responsible for annual and final reports. In the absence of the chair, the vice-chair will perform these duties. The secretary will record and distribute the minutes of the meetings.
Literature Cited
Ahuja, L. R., K. W. Rojas, J. D. Hanson, M. J. Shaffer, and L. Ma, 2000. The Root Zone Water Quality Model. Highlands Ranch, Colo.: Water Resources Publications.
Ajayi, A. E. and A. A. Olufayo. 2004. Evaluation of two temperature stress indices to estimate grain sorghum yield and evapotranspiration. Agron. J. 96, 1282–1287.
Al-Jamal, M. S., T. W. Sammis, and T. Jones. 1997. Nitrogen and chloride concentration in deep soil cores related to fertilization. Agricultural Water Management. 34: 1-16.
Allen, R. G., L. S. Pereira, D. Raes, and M. Smith. 1998. Crop evapotranspiration, guidelines for computing crop water requirements. FAO Irrig. and Drain. Paper 56. Food and Agric. Organ. United Nations, Rome, Italy. 300 pp.
Allison, F. E. 1996. The fate of nitrogen applied to soils. Advances in Agronomy. 18, 219-258.
Andrade, M. S. Evett, and S. O’Shaughnessy. 2018. Machine Learning Algorithms Applied to the Forecasting of Crop Water Stress Indicators. In Proceeding of the 2018 Irrigation Association Technical Conference.
Ayars, J. E., C. J. Phene, R. B. Hutmacher, K. R. Davis, R. A. Shoneman, S. S. Vail, R. M. Mead. 1999. Subsurface drip irrigation of row crops: a review of 15 years of research at the Water Management Research Laboratory. Agric. Water Mgt. 42:1-27.
Bouyoucos, G. T. 1915. Effect of temperature on the movement of water vapor and capillary moisture in soils. J. Agric. Res. 5:141–172.
Broner, I. 2005. Irrigation scheduling. In Service in Action; No. 4.708; Colorado State University Extension: Fort Collins, CO, USA.
Bucks, D. A., L. J. Drie, and O. F. French. 1974. Quality and frequency of trickle and furrow irrigation for efficient cabbage production. Agronomy Journal. 66: 53-56.
Camp, C. R. 1998. Subsurface drip irrigation: A review. Trans. ASAE. 41(5)1353-1367.
Camp, C. R., F. R. Lamm, R. G. Evans, and C. J. Phene. 2000. Subsurface drip irrigation: Past, present and future. In proc. of the 4th Decennial Nat’l Irrigation Symp., Phoenix, AZ, Nov. 14-16. pp. 363-372.
Campillo C, M. H. Prieto, C. Daza, M. J. Monino, M. Garcia, I. Goodwin, and M. G. O’Connel. 2008. Use of stem water potential measurements to correct crop water needs estimations in a rotation of two horticultural crops. Acta Horticulturae 72:147-154.
Cardenas-Lailhacar, B. and Dukes, M. 2010. Precision of soil moisture sensor irrigation controllers under field conditions. Agric. Water Manag. 2010, 97, 666-672.
Casanova, J. J., S. R. Evett, R. C. Schwartz. 2012. Design and field tests of an access-tube soil water sensor. Appl. Eng. Agric. 28(4):603-610).
Cepuder, P. and M. K. Shukla. 2002. Groundwater nitrate in Austria: A case study in Tullnerfeld. Nutrient Cycling in Agro ecosystems. 64: 301-315.
Colaizzi, P. D., E. M. Barnes, T. R. Clarke, C. Y. Choi, and P. M. Waller. 2003. Estimating soil moisture under low-frequency surface irrigation using Crop Water Stress Index. J. Irrig. Drain. Eng. 129, 27–35.
Conejero W., C. D. Mellisho, M. F. Ortuno, A. Galindo, F. Perez-Sarmiento and A. Torrecillas. 2011. Establishing maximum daily trunk shrinkage and midday stem water potential reference equations for irrigation scheduling of early maturing peach trees. Irrigation Science 29(4): 299-309.
Deb S. K., M. K. Shukla, P. Sharma, and J. Mexal. 2011. Coupled liquid water, water vapor, and heat transport simulations in an unsaturated zone of a sandy loam field. Soil Science. 176(8):387-398.
Deb S. K, P. Sharma, M. K. Shukla and T. W. Sammis. 2013a. Drip-irrigated pecan seedling response to irrigation water salinity. Hort. Science. 48:1-8.
Deb, S. K., M. K. Shukla, J. Simunek, and J. G. Mexal. 2013b. Evaluation of spatial and temporal root water uptake patterns of a flood-irrigated pecan tree using the HYDRUS (2D/3D) model. ASCE, Irrigation and Drainage Engineering. 139: 599-611.
Deb, S. K., P. Sharma, M. K. Shukla, J. Ashigh, and J. Šimůnek. 2015. Numerical Evaluation of Nitrate Distributions in the Onion Root Zone under Conventional Furrow Fertigation. J. Hydrol. Eng. 21(2): 05015026-1. doi:10.1061/(ASCE)HE.1943-5584.0001304.
Ebrahimian, H., A. Liaghat, M. Parsinejad, E. Playan, F. Abbasi, M. Navabian, and B. Lattore. 2013. Optimum design of alternate and conventional furrow fertigation to minimize nitrate loss. J. Irrig. Drain. Eng. 139:911-921. doi:10.1061/(ASCE)IR.1943-4774.0000635.
Evett, S., and J. Steiner. 1995. Precision of neutron scattering and capacitance type soil water content gauges from field calibration. Soil Sci. Soc. America J. 59(4): 961-968.
Evett, S. R., R. C. Schwartz, J. A. Tolk, and T. A. Howell. 2009. Soil profile water content determination: Spatiotemporal variability of electromagnetic and neutron probe sensors in access tubes. Vadose Zone J. 8(4): 926-941.
Evett, S., H. Schomberg, A. Thompson, R. Schwartz, S. O’Shaughnessy, and M. Andrade. 2018. Water in the Cloud: A New System for Field Water Monitoring With Cloud Data Access. In Proceeding of the 2018 Irrigation Association Technical Conference.
Fares, A. 2013. Water Management Software to Estimate Crop Irrigation Requirements for Consumptive Use Permitting In Hawaii: Version 2.0. Final Report for the Commission on Water Resource Management, Hawaii Department of Land and Natural Resources. Final Report.
Fisher, D.K., Hanks, J.E., and Pringle, H.L., III. 2009. Comparison of Irrigation Scheduling Methods in the Humid Mid-South. Irrigation Association. 2009.
Garnier E and A. Berger. 1985. Testing water potential in peach trees as an indicator of water stress. J. Horticultural Science, 60, 47-56.
Girona J, M. Mata, J. del Campo., A. Arbones, E. Bartra, and J. Marsal. 2006. The use of midday leaf water potential for scheduling deficit irrigation in vineyards. Irrigation Science 24(2): 115-127.
Gowda, P., T. A. Howell, J. R. Ennis, T. H. Marek, and D. O. Porter. 2011. Bushland, Texas Reference ET Calculator [abstract]. ASA-CSSA-SSSA Annual Meeting Abstracts. Paper No. 68-7.
Gustafson, C. D. 1975. Drip Irrigation-Worldwide 1975, Present Status and Outlook for Drip Irrigation. Survey Report of University of California, San Diego, CA, pp. 1-5.
Halvorson A. D., F. C. Schweissing, M. E. Bartolo, C. A. Reule, and A. Berrada. 2008. Nitrogen effects on onion yield under drip and furrow Irrigation. Agronomy Journal.. 100: 1062-1069.
Hanks, R. J., H. R. Gardner, and M. L. Fairbourn. 1967. Evaporation of water from soils as influenced by drying with wind and radiation. Proc. Soil Sci. Soc. Am. 31:593–598.
Hanson, B. R., J. Šimůnek, and J. W. Hopmans. 2006. Numerical modeling of urea-ammonium-nitrate fertigation under microirrigation, Agric. Water Management, 86, 102-113, 2006.
Hoffman, G. J., T. A. Howell, and K. H. Solomon. 1990. Management of farm irrigation systems. ASAE Monograph. ASAE, St. Joseph MI. 1015 pp.
Irmak, S., D. Z. Haman, and R. Bastug. 2000. Determination of crop water stress index for irrigation timing and yield estimation of corn. Agron. J. 92, 1221–1227.
Jackson, R. D., S. B. Idso, R. J. Reginato, and P. J. Pinter. 1981. Crop canopy temperature as a crop water stress indicator. Water Resour. Res. 17, 1133–1138.
Jackson, T. and T. Schmugge. 1989. Passive microwave remote sensing system for soil moisture: Some supporting research. IEEE Trans. on Geoscience and Remote Sensing 27(2): 225-235.
Jones, H. 2004. Irrigation scheduling: advantages and pitfalls of plant-based methods. J. Exp. Bot. 55, 2427–2436.
Kalma, J. D and D .L. B. Jupp. 1990. Estimating evaporation from pasture using infrared thermometry: evaluation of a one-layer resistance model. Agric. Forest Metero. 51, 223–246.
Kandelous, M.M., and J. Šimůnek. 2010. Comparison of numerical, analytical and empirical models to estimate wetting pattern for surface and subsurface drip irrigation. Irrig. Sci. 28:435-444. doi:10.1007/s00271-009-0205-9.
Kebede, H., Fisher, D.K., Sui, R., and Reddy, K.N. 2014. Irrigation Methods and Scheduling in the Delta Region of Mississippi: Current Status and Strategies to Improve Irrigation Efficiency. Am. J. Plant Sci. 2014, 5, 2917-2928.
Kisekka, I. 2019. Personal communication, January 2019.
Kisekka, I., T. Oker, G. Nguyen, J. Aguilar, and D. Rogers. 2017. Revisiting precision mobile drip irrigation under limited water. Irrigation Science. 35: 483 https://doi.org/10.1007/s00271-017-0555-7
Kustas, W. P. and J. M. Norman. 1997. A two-source approach for estimating turbulent fluxes using multiple angle thermal infrared observations. Water Resour. Res. 33, 1495–1508.
Lamm, F. R. 2016. Cotton, Tomato, Corn, and Onion Production with Subsurface Drip Irrigation - A Review. Trans. ASABE Vol. 59(1):263-278.
Lamm, F. R. and C. R. Camp. 2007. Subsurface drip irrigation. Chapter 13 in Microirrigation for Crop Production - Design, Operation and Management. F.R. Lamm, J.E. Ayars, and F.S. Nakayama (Eds.), Elsevier Publications. pp. 473-551.
Lamm, F. R. and T. P. Trooien. 2003. Subsurface drip irrigation for corn production: A review of 10 years of research in Kansas. Irrig. Sci. 22(3-4):195-200.
Lamm, F. R., J. E. Ayars, and F. S. Nakayama (Eds.). 2007. Microirrigation for Crop Production - Design, Operation and Management. Elsevier Publications. 608 pp.
Lamm, F. R., D. M. O’Brien, and D. H. Rogers. 2015. Economic comparison of subsurface drip and center pivot sprinkler irrigation using spreadsheet software. Applied Engineering in Agric. 31(6):929-936.
Levy, Y., R. H. Shapira, B. Chefetz, and D. Kurtzman. 2017. Modeling nitrate from land surface to wells' perforations under agricultural land: success, failure, and future scenarios in a Mediterranean case study. Hydrol. Earth Syst. Sci. 21:3811-3825. doi: 10.5194/hess-21-3811-2017.
Martin, E. 2009. Methods of Measuring for Irrigation Scheduling-WHEN; Arizona Cooperative Extension: Tucson, AZ, USA, 2009.
Martin, E.C., Pegelow, E.J., and Stedman, S. 1995. Comparison of Irrigation Scheduling Methods in Cotton Production; College of Agriculture, University of Arizona: Tucson, AZ, USA.
Mazahrih, N., N. Katbeh-Bader, S. Evett, J. Ayars, and T. Trout. 2008. Field calibration accuracy and utility of four down-hole water content sensors. Vadose Zone J. 7(3): 992-1000.
McCown, R. L. G. L. Hammer, J. N. G. Hargreaves, D. P. Holzworth, and D. M. Freebairn. 1996. APSIM: A Novel Software System for Model Development, Model Testing and Simulation in Agricultural Systems Research. Agricultural Systems 50 (1996) 255-271.
McCutchan, Harold and K.A. Shackel. 1992. Stem-water potential as a sensitive indicator of water stress in prune trees (Prunus domestica L. cv. French). Journal of the American Society for Horticultural Science 117(4):607-611.
Merriam, J. 1966. A management control concept for determining the economical depth and frequency of irrigation. Trans. ASAE 9(4): 492-498.
Miyamoto, S., and J. B. Storey. 1995. Soil management in irrigated pecan orchards in the southwestern United States. HortTechnology 5:219–222.
Nassar, I. N. and R. Horton. 1992. Simultaneous transfer of heat, water, and solute in porous media: I. Theoretical development. Soil Sci. Soc. Am. J. 56:1350–1356.
Nakayama, F. S. and D. A. Bucks, Eds. 1986. Trickle Irrigation for Crop Production - Design, Operation and Management. Elsevier Publications. 383 pp.
O’Shaughnessy, S. A., and S. R. Evett. 2010a. Developing wireless sensor networks for monitoring crop canopy temperature using a moving sprinkler system as a platform. Applied Eng. in Agric. 26(2), 331-341.
O’Shaughnessy, S. A., and S. R. Evett. 2010b. Canopy temperature based system effectively schedules and controls center pivot irrigation for cotton. Agric. Water Mgmt. 97(9), 1310-1316.
O’Shaughnessy, S. A., M. A. Hebel, S. R. Evett, and P. D. Colaizzi. 2011. Evaluation of a wireless infrared thermometer with a narrow field of view. Comput. Electron. Agric. 76(1), 59-68.
Peters, R. T. and S. R. Evett. 2007. Spatial and temporal analysis of crop stress using multiple canopy temperature maps created with an array of center-pivot-mounted infrared thermometers. Trans. ASABE 50, 919–927.
Peters, R. T., and S. R. Evett. 2008. Automation of a center pivot using the temperature-time-threshold method of irrigation scheduling. J. Irrig. Drainage Engr. 134 (3), 286-290.
Peterson, G. A. and J. F. Power. 1991. Soil, crop, and water management. In: Managing nitrogen for groundwater quality and farm profitability, R. F. Follett et al., Eds., pp. 189–198, SSSA, Madison, WI.
Phene, C. J. 1995. Research trends in microirrigation. In: Microirrigation for a changing world. Proc 5th Int’l. Microirrigation Congress. F. R. Lamm, (Ed.), St. Joseph, Michigan. ASAE. pp. 6-24.
Richards, L. A. 1931. Capillary conduction of liquids through porous media. Physics 1:318–333.
Rogers, D. H. 2012. Introducing the Web-based Version of KanSched: An ET-based Irrigation Scheduling Tool. In: Proc. 24th annual Central Plains Irrigation Conference, Colby, Kansas, Feb. 21-22, 2012. Available from CPIA, 760 N.Thompson, Colby, KS. pp. 230-236.
Ross, P. J., J. Williams, and R. L. McCown. 1985. Soil temperature and the energy balance of vegetative mulch in the semi–arid tropics: II. Dynamic analysis of the total energy balance. Aust. J. Soil Res. 23:515–532.
Saito, H., J. Simunek, and B. P. Mohanty. 2006. Numerical analysis of coupled water, vapor, and heat transport in the vadose zone. Vadose Zone J. 5:784–800.
Scanlon B. R. and P. C. D. Milly. 1994. Water and Heat Fluxes in Desert Soils 2. Numerical Simulations. · Water Resources Research 30(3):721-734.
Schwartz, R. C., S. R. Evett, and J. M. Bell. 2009. Complex permittivity model for time domain reflectometry soil water content sensing: II. Calibration. Soil Sci. Soc. America J. 73(3): 898-909.
Shackel K. A. 2011. A Plant-based Approach to Deficit Irrigation in Trees and Vines. Hort.Sci. 46:173-177.
Shaffer, M.J., A.D. Halvorson, and F.J. Pierce. 1991. Nitrate leaching and economic analysis package (NLEAP): model description and application. In: Follett RF, Deeney DR, Cruse RM (eds) Managing nitrogen for groundwater quality and farm profitability. Soil Sci. Soc. Am. J. Inc., Madison, Wisconsin, pp 285-298.
Šimůnek, J. 2019. Personal communication, January 2019.
Šimůnek, J., M. Th. van Genuchten, and M. Šejna. 2016. Recent developments and applications of the HYDRUS computer software packages, Vadose Zone Journal, 15(7), pp. 25, doi: 10.2136/vzj2016.04.0033, 2016.
Šimůnek, J., M. Šejna, and M. Th. van Genuchten. 2018. New features of the version 3 of the HYDRUS (2D/3D) computer software package, Journal of Hydrology and Hydromechanics, 66(2), 133-142, doi: 10.1515/johh-2017-0050, 2018.
Sinclair, T. R. and M. M. Ludlow. 1985. Who taught plants thermodynamics? The unfulfilled potential of plant water potential. Aust. J. Plant Physiol. 12:213-217.
Sui, R. 2017. Irrigation Scheduling Using Soil Moisture Sensors. J. Agric. Sci. 2017, 10, 1.
Taylor, S. A. and L. Cavazza. 1954. The movement of soil moisture in response to temperature gradients, Soil Sci. Soc.Amer. Proc., 18, 351-358.
Topp, G., J. Davis, and A. Annan. 1980. Electromagnetic determination of soil water content: Measurements in coaxial transmission lines. Water Resources Res. 16(3): 574-582.
Wescot, D. W. and P. J. Wierenga. 1974. Transfer of Heat by Conduction and Vapor Movement in a Closed Soil System. Soil Sci. Amer. J. 38(1)9-14.
Wichelns, D. 2007. Economic implications of microirrigation. Chapter 16 in Microirrigation for Crop Production - Design, Operation and Management. F.R. Lamm, J.E. Ayars, and F.S. Nakayama (Eds.), Elsevier Publications. pp. 221-258.
Yoder, R.; Johnson, D.; Wilkerson, J.; and Yoder, D. 1998. Soilwater sensor performance. Appl. Eng. Agric. 14, 121-133.
Zotarelli, L., Scholberg, J.M., Dukes, M.D., Muñoz-Carpena, R., and Icerman, J. 2009. Tomato yield, biomass accumulation, root distribution and irrigation water use efficiency on a sandy soil, as affected by nitrogen rate and irrigation scheduling. Agric. Water Manag. 2009, 96, 23-34.