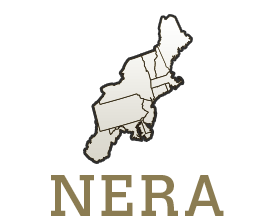
NE138: Epidemiology and Control of Emerging Strains of Poultry Respiratory Disease Agents
(Multistate Research Project)
Status: Inactive/Terminating
NE138: Epidemiology and Control of Emerging Strains of Poultry Respiratory Disease Agents
Duration: 10/01/1996 to 09/30/2001
Administrative Advisor(s):
NIFA Reps:
Non-Technical Summary
Statement of Issues and Justification
Respiratory disease continues to be a major cause of economic losses in the poultry industry. Diseases such as infectious bronchitis, avian influenza, infectious laryngotracheitis and avian mycoplasms are generally the outcome of complex interactions. Variable causative agents, immunosuppression, host susceptibility, and the influence of the environment effect the epizootic manifestation of respiratory disease. Understanding the pathogenesis and epizootic manifestations of respiratory disease is crucial for development of control strategies. Reduced use of antibiotics and antimicrobials has led to increased pressure on the industry to rely more heavily on other methods of disease, such as the use of vaccines as well as the more long range alternatives such as antivirals. The application of molecular and conventional research approaches is needed to identify, diagnose, and control emerging respiratory pathogens. This multi-disciplinary approach requires the expertise and collaboration of virologists, bacteriologists, immunologists, pathologists and molecular biologists.
Related, Current and Previous Work
A CSREES search was conducted and showed several projects with objectives involving the agents studied in this project. The majority of the projects currently being conducted are with researchers listed in this project. It is the intent of our group to expand our participation with other researchers over the project period by continuing to interact with colleagues working with these agents. For instance, members of the North Central 116 (NC-116) regional project working with turkey influenza virus at Minnesota AES could easily conduct work under this project's objectives. The joint meeting with the NC-116 group in Chicago will serve to assist us in project recruitment and collaboration (see ORGANIZATION section).
A. Infectious Bronchitis Virus (IBV)
Infectious bronchitis (IB) is a highly contagious disease of chickens that causes significant economic losses in commercial broilers, layers and broiler breeders. The many different recognized serotypes and emerging variant serotypes (Gelb et al., 1991) make IB among the most difficult poultry diseases to control. Variant IBV's pose a major threat to commercially raised poultry throughout the world. In the U.S. in 1992, serious yet unrelated outbreaks occurred in broilers in the Delmarva peninsula area, caused by the Delaware variant (92-072), and the Southeastern region caused by the Arkansas serotype and the closely-related Georgia variant. Although recognized since the late 1980's in the Central Valley region, the California variant still causes considerable losses in broilers. IBV causes acute respiratory disease that is commonly followed by secondary bacterial infection most often due to Escherichia coli, particularly in broiler chickens. The combination often results in elevated growing mortality and airsacculitis condemnation rates in affected flocks. Layers and broiler breeders also may be highly susceptible to variant IB. Egg production and losses are common particularly on farms with multiple-aged flocks. Layer and broiler flocks infected with pathogenic mycoplasma experience more severe IBV-related egg production and respiratory disease associated losses. The high incidence of IBV infections in commercial chickens underscores the importance of developing vaccination programs that afford maximal protection under field conditions.
IB is perhaps the most "explosive" of all avian diseases. The virus spreads extremely rapidly in flocks due to its short incubation period (18-48 hours) and highly contagious nature (King and Cavanagh, 1991). The virus is present in respiratory excretions and feces and is disseminated by aerosol transmission. Mechanical transmission of the virus by humans on contaminated clothing and poultry equipment can be very important in IB spread.
IBV enters the chicken by inhalation or ingestion of contaminated feed or drinking water and replicates initially in the upper respiratory tract. Viral damage to respiratory tissues includes deciliation of tracheal epithelial cells and impairment of the production and secretion of mucus from glands lining the trachea ultimately resulting in predisposition to secondary bacterial infections. Pneumonia involving the bronchial and pleural surfaces of the lung may occur with more virulent strains and result in higher mortality. Virus is also disseminated to enteric tissues, such as proventriculus, intestine and cecal tonsil (Ambali and Jones, 1990; Gelb, 1989), as well as kidney (Gumming, 1969), and oviduct (Crinion and Hofstad, 1972). Damage to the oviduct produces egg production losses in the form of a cessation or decrease of egg production and an increase in egg quality problems (wrinkled shells and lost pigmentation, watery albumin). Virus is shed from the cecal tonsil and is present in feces for several weeks or months after the acute disease episode (Cook, 1968; Alexander and Gough, 1977). Cellular tropism of mammalian coronaviruses can be correlated to their virulence and in vivo persistence (Fleeting et al.,1994; Stuhler et a1.,1994).
Immunity following recovery from natural infection or live IBV vaccination is of variable duration and specificity depending on the virulence of the field or vaccine strain, age of infection or vaccination, immunocompetency of the flock, the presence of potentially interfering maternal antibodies, and the serotype and virulence of a subsequent challenge strain. Vaccinal protection results following the application of IBV live attenuated strains) onto the respiratory mucosal surface by the aerosol (spray), eyedrop, or drinking water (exposure via the palatine cleft) routes. Vaccines given by these "natural" routes of application mimic the natural portal of infection and induce local tissue immune responses in the upper respiratory tract. On the other hand, inactivated oil emulsion vaccines given by injection do not stimulate significant respiratory immunity, but are effective in stimulating systemic blood-borne resistance in live IB V-primed birds by providing protection to the internal target tissues, kidney and oviduct.
Immunosuppression associated with IBDV infection, particularly during the first two weeks of life, is known to increase the severity of viral respiratory diseases, contribute to prolonged shedding of live Newcastle disease virus (NDV) and IBV, as well as impair the response to vaccination with NDV, laryngotracheitis virus, and IBV (Allan et al., 1972; Faragher et al., 1974; Pattison and Allan, 1974; Rosenberger and Gelb, 1978). Research has also shown that IBDV destroys local antibody-producing plasma cells in the gland of Harder, and thus may directly compromise upper respiratory tract immunity (Dohms et al., 1981). Field observations over the years have also clearly demonstrated the relationship between early (< 2 week) infection in broilers and poor flock performance in terms of elevated airsacculitis condemnations and a greater incidence and severity of respiratory disease challenges and mortality. As a result, much effort is directed at protecting baby chicks from the immunosuppressive effects of IBDV infection. The impact of early infection has been reduced by providing passive protection (IBDV maternal antibody) to chicks through the use of IBDV immunization programs in breeder flocks. This strategy has been successful in preventing early infection and long-lasting immunosuppression, however it does not prevent infection after maternal immunity wanes at 2-4 weeks of age. In fact, infection at 2-4 weeks produces a more transient immunosuppression than ore-day infection but still has the potential to impact respiratory disease immunity. IBDV infection of 3-week broiler chickens produced plasma cell necrosis in the gland of Harder, from 5-14 days after inoculation and reduced antibody production to sheep red blood cells and Brucella abortus antigens (Dohms et al., 1988; Dohms and Jaeger, 1988).
Diagnosis of IBV is accomplished by isolating and serotyping the causative field isolate. The virus-neutralization (VN) and hemagglutination-inhibition (HI) tests have been until very recently the only procedures used for serotyping (Gelb, 1989). These procedures are expensive, tedious, and time-consuming and are not widely available to the poultry industry. Serodiagnosis of IBV using serum from recovered flocks can not be used to identify the causative serotype of IBV because chickens produce cross-reacting antibodies following multiple infections (Gelb and Killian, 1987). Serotype-specific monoclonal antibodies (Mabs) developed for the vaccinal serotypes, Mass, Ark, and Conn have been used to identify vaccinal serotype(s) involved in IB outbreaks (Karaca et al., 1992). The polymerase chain reaction (PCR) has been used successfully to diagnose viral infections in humans and animals (Andreasen et al., 1991; Homberger et al., 1991). The technique is particularly attractive in that it is extremely sensitive and is capable of detecting minute amounts of virus in clinical specimens. The PCR is also highly specific in that it utilizes oligonucleotide primers that potentially may recognize specific gene sequences that encode important epitopes of viral proteins responsible for initiating infection through attachment and inducing protective immunity. In addition, the PCR is a rapid test giving results in a matter of days. PCR is currently used for the diagnosis of IBV serotypes. Two reverse transcritption (RT) PCR approaches have been used to identify IBV serotypes. Restriction fragment length polymorphism (RFLP) was developed by Kwon et al. (1993). Alternatively, RT PCR using serotype-specific primers has been used to identify IBV (Reed et al., 1992).
The structure and replication of IBV and other coronaviruses have been reviewed (Boursnell et al., 1989; Spaan et al., 1988). The virion has a helical symmetry and is 80-160 nm in diameter. The ss RNA 27.6 kb IBV co-linear genome is arranged into six regions, each containing one or more open reading frames which are separated by intergenic sequences containing signals for transcription of multiple subgenomic m-RNAs. Genomic RNA is of a positive polarity and as such serves as a template for the production of full-length negative strand RNA. The 5' and 3' ends of the genome contain noncoding homology sequences that on the 3' end have been proposed to serve as a RNA polymerase binding site for production of the negative strand (Boursnell et al., 1989). The negative strand serves as template for the production of genomic RNA and subgenomic m-RNAs. Subgenomic m-RNAs A (nucleocapsid), B, C (membrane), D, E (spike), and F (RNA polymerise) form a 3' coterminal nested set that are structurally multicistronic but are functionally monocistronic. Only the gene located at the 5' end of each m-RNA is translated. These m-RNAs are synthesized m constant but nonequimolar concentrations by a unique leader-primed transcription process. Analysis of the 5' end of genomic and m-RNAs has revealed a common leader sequence, CUUAACAA (Brown and Boursnell, 1984) or the very similar CUGAACAA (Boursnell et al., 1987). Leader sequences of about 60-65 nucleotides are transcribed discontinuously from the 3' end of the negative strand template by viral RNA polymerise. The conserved (i.e., homology) sequences are thought to be the initiation sites .for transcription by the leader-RNA polymerise complex. The degree of complementarity (basepairing) between the free leader and the different initiation sites may regulate the expression of the m-RNAs. The core homology is conserved in all the intergenic regions, however the region around the core is not conserved for different genes even in a single IBV serotype. When the intergenic region for the matrix gene was compared for several Mass strains and four other European serotypes, including some recently isolated variants, high homology was found around the identical core homology (Cavanagh and Davis, 1988).
The virus possesses three major structural proteins; a 45kD nucleocapsid protein (N), a (23kD) membrane or matrix (M) glycoprotein and an envelope spike (S) glycoprotein peplomer. The N protein is involved in encapsidation of genomic RNA and has been implicated in the process of RNA replication (Spain et al., 1988). The M protein is required for viral maturation and assembly interacting with N and perhaps genomic RNA. The S gene encoding for subunits S-1 (gp 92) and S-2 (gp 84) has received considerable attention since S-1 is required for virus attachment to host cells and erythrocytes (Cavanagh and Davis, 1986) and the induction of immunity (Cavanagh et al., 1986;1988). Sequence analysis of the S-1 gene of IBV has identified hypervariable regions characterized by nucleotide substitutions, sequence insertions and deletions that are not conserved among serotypes (Banns et al., 1986; Kusters et al., 1989). The hypervariable sequences in S-1 have been mapped to regions encoding serotypic determinants (Cavanagh et al., 1988; Kusters et al., 1989).
Non-structural proteins encoded by the coronavirus genome include an RNA polymerise (mRNA F ) used in the synthesis of genomic RNA and m-RNAs. The RNA polymerise, however has yet to be identified. In addition, coronavirus infected cells have two m-RNAs (B and D) for which proteins have yet to be identified. m-RNA B potentially encodes two proteins (MW 7.5 and 9.5 Kd) and mRNA D may encode three proteins (MW 6.7, 7.4, and 12.4 Kd). The 12.4 Kd protein has been detected in IBV infected chicken embryo kidney cells by immunoprecipitation and is thought to be virion associated (Liu et al., 1991). The other two open reading frames associated with mRNA D have been transcribed in vitro (Liu et al., 1991). The roles) of these mRNAs or proteins is not known (Spain et al., 1988).
Antisense oligonucleotides (anti-oligos) have been used to study gene function by blocking gene expression in eucaryotic and procaryotic systems (Stein and Cheng, 1993). Anti-oligos have been used to selectively inhibit DNA and RNA viral gene expression and replication (Agrawal, 1992). The technology provides an useful alternative to standard (homologous recombination) mutational analysis which is difficult with large RNA viruses like coronaviruses. Anti-oligos inhibit viral replication by blocking translation through binding to viral m-RNA complementary bases (Stephenson and Zamecnik, 1978; Vickers et al., 1991). Effective anti-oligos have been directed at the 5' non-coding translation initiation sites of several viruses (Gupta, 1987; K. C., 1987; LeMaitre et al., 1987; Offensperger et al., 1993; Storey et al., 1991). Alternatively, transcription may also be affected through base pairing with the genomic RNA serving as a template for the replication of virion RNA (LeMaitre et al., 1987). Specific antiviral activity has been reported for human and avian retroviruses (HIV, Rous sarcoma virus, HTLV III), herpesviruses (HSV), rhabdoviruses (VSV), influenza virus, and papilloma virus (Agrawal, 1992). The antiviral chemotherapeutic potential of anti-oligos in vivo was demonstrated in poultry using duck hepatitis virus (Offensperger et al., 1993).
The stability and efficiency of uptake of exogenously-applied anti-oligos by cultured cells has been studied. Phosphodiester oligodeoxyribonucleotides maybe degraded by nucleases (Thierry and Dritshilo, 1992). Nuclease-resistant phosphorothioate and methylphosphonate analogs have been extensively used (Hoke et al., 1991;Storey et al. 1991). Concentrations of antioligos required for antiviral activity range from uM for phosphorothioate analogs (Vickers et al., 1991) to nM for poly-L-lysine-conjugated anti-oligos (LeMaitre et al., 1987). Uptake of oligomers fom 10-25 nucleotides in length may occur spontaneously or be facilitated by liposomes (Thierry and Dritshilo, 1992).
B. Infectious laryngotracheitis virus (ILTV)
Infectious laryngotracheitis virus (ILTV), an herpesvirus causes an acute respiratory disease (ILT) of world-wide importance to the poultry industry. Twice in the last ten years severe outbreaks of the disease have occurred on the Delmarva peninsula, causing severe financial losses to the poultry industry. In 1994/1995, the Delmarva peninsula and other areas of the southeastern United States experienced a serious epornitic of ILT. Approximately 370 cases were submitted to the University of Delaware's Poultry Diagnostic Laboratory from September 1994 through June of 1995. There were an additional 185 cases reported in Georgia in 1995. The disease was also reported in Alabama and Arkansas. Fortunately, ILT is a slowly spreading, controllable disease, which can cause high mortality and condemnation. Unfortunately, the presence of ILT prevent the export of U.S. poultry to some foreign markets.
There is considerable interest in developing safe and easily monitored ILTV vaccines. Vaccination has generally been used only in areas where the disease is endemic, since vaccination can result in the occurrence of long term "carrier birds" due to the virus' ability to enter a latent state. Furthermore, current vaccines are themselves mildly pathogenic, with a resulting economic "cost". There is justifiable concern over the negative performance (growth, mortality, feed conversion) associated with current ILT vaccines. It is thought that present live virus vaccines may mutate and become responsible for disease outbreaks. A series of reports by Guy et al. (1989, 1990, 1991) suggested that vaccine viruses played a role in outbreaks of ELT and that vaccine viruses increased in virulence after bird-to-bird passage. In contrast, another group (Hastings, 1991; Keeler et al., 1993) has proposed that ILTV vaccine strains are genetically stable and that the inability to differentiate vaccine strains from field isolates is due to the limitations of restriction fragment length polymorphisms (RFLPs) analysis.
Infectious laryngotracheitis was first described in 1925 (May and Tittsler, 1925). In its acute form, ILT is characterized by signs of respiratory distress in birds, accompanied by gasping and expectoration of bloody exudate (Hanson, 1991). In addition, the mucous membranes of the trachea become swollen and hemorrhagic. The epizootic form of the disease spreads rapidly and can affect up to 90-100% of an infected flock. Mortality generally averages between 10-20%. Milder forms of the disease are characterized by watery eyes, conjunctivitis, persistent nasal discharge and a reduction in egg production (Cover and Benton, 1958).
Several groups have tried to differentiate strains of ILTV on the basis of RFLPs. In the U. S., Andreasen et al. (1990), Guy et al. (1989), Keeler et al. (1993 ) and Keller et al. (1992) have reported that most field isolates of ILTV have restriction patterns which are identical or very similar to those observed for embryo-propagated ILTV vaccine strains. However, with the exception of Guy et al. (1989), the other three groups reported the presence of field isolates of ILTV that exhibited unique restriction patterns. The general similarity in ILTV DNA restriction patterns tends to confuse the issue regarding the source of virus resulting in field outbreaks of ILT.
The avian immune response to ILTV has been extensively studied. There is no evidence that neutralizing antibodies correlate with protection. A prominent role of cell-mediated immunity has been suggested. Chickens surgically bursectomized at one-day of age and subsequently treated with cyclophosphamide resisted virulent ILTV challenge without the presence of mucosal antibody (Fahey et al., 1983; Fahey and York, 1990). In addition, adoptive transfer of spleen cells from immune donors conferred protection (Fahey et al., 1984). Furthermore, recent experiments using inbred lines of chickens have also suggested an association of the chicken MHC with ILTV resistance (Loudovaris et al., 1991a; Loudovaris et al., 1991b). However, it is not known whether protection is mediated directly by cytotoxic T-lymphocytes (CTL), by soluble factors released by activated T-cells, or a combination of the above. It is also not known what components of the mature virion are responsible for eliciting this protective immune reaction.
ILTV contains a linear, double-stranded DNA genome approximately 155 kilobase pairs (kb) in length (Bagust and Johnson, 1995; Kotiw et al., 1982; Johnson et al., 1991). ILTV exhibits the physical characteristics of class 2 herpesviruses such as pseudorabies virus (PRV) and equine herpesvirus 1 (EHV-1). In the last five years, a number of ILTV genes have been identified and sequenced. For a review see Bagust and Johnson, 1995. The University of Delaware laboratory has identified the thymidine kinase, glycoprotein B, glycoprotein C, and glycoprotein H gene homologues in the unique long region of the genome, a SORF3 gene homologue in the inverted repeat region, and nine unique short region genes (Keeler et al., 1991; Kingsley et al., 1994; Poulsen et al., 1991).
The host range of ILTV is limited to the chicken. Non-avian cell lines cannot be used to propagate the virus. ILTV grows in chicken embryos and chicken cell cultures. Fortunately, within the past few years, two groups have reported the ability to adapt ILTV to grow on continuous avian liver cell lines (Schnitzlein et al., 1994; Scholz et al., 1993).
C. Avian Influenza Virus (AM
Avian influenza (AI) is a disease associated with several clinical syndromes and infected chickens exhibit various clinical signs. AI virus (AIV) isolates can be categorized into 14 hemagglutinin (H1-14) and 9 neuraminidase (N1-9) subtypes (Easterday and Hinshaw, 1991; Kawaoka et a1 1990). In most birds species, especially free-living or captive wild bird species of the orders Anseriformes (migratory waterfowl) and Charadriformes (shorebirds), AIV infections are asymptomatic (Slemons et al., 1974; Stallknecht and Shane, 1988). Waterfowl and shore birds are considered to be primary reservoirs for AIVs. Recently, ratites have been shown to harbor AIV and could serve as a source of infection of commercial poultry. In poultry, AI is frequently subclinical, but can be associated with mild to moderately severe clinical disease syndromes such as respiratory disease, decreases in egg production and renal disease, especially in commercial poultry (Alexander and Gough, 1986; Alexander et al., 1981; Halvorsen et al., 1980; Johnson and Maxfield, 1976; Selleck et al., 1994; Skeeles et al., 1974). Typically, these diseases have been accompanied by low mortality and were self-limiting, but in some outbreaks, the mortality has been high, especially when accompanied by exacerbating factors such as concurrent infections, physiologic stresses or certain environmental factors (Newman et al., 1981).
Since 1950, 13 outbreaks of HS or H7 AIVs have been associated with severe systemic disease in chickens and turkeys with accompanying high morbidity and mortality (Easterday and Hinshaw, 1991). These diseases were historically termed fowl plague, but the more accurate and current designation is highly pathogenic (HP) AI (Bankowski, 1981). Such viruses express high lethality in pathogenicity tests for chickens.
No avian or mammalian reservoir for HPAIVs has been identified. By contrast, epidemiologic and molecular genetic information suggests that HPAIVs arise via mutation of nonpathogenic (NP) or mildly pathogenic (MP) AIVs. For example, in the 1983-84 outbreak of H5N2 AIV in the northeastern U.S., the disease began as an acute respiratory syndrome associated with low mortality and reduced egg production (Eckroade and Silverman-Bachin, 1986). Initial virus isolates were categorized as NP AIV based on failure to kill 4-week-old chickens in laboratory pathogenicity tests. Six months later, the disease had changed character to become a severe systemic syndrome producing total cessation of egg production within a few days, SO-90% mortality and nervous signs in affected chickens. These new isolates were experimentally pathotyped as HP. Analysis of the hemagglutinin of AIVs from Pennsylvania identified the loss of a glycosylation site at amino acid residue 13 as being associated with a change from NP-MP to HP (Kawaoka et a1.,1984). Subsequently, emergence of HP variants from AIVs of lower pathogenicity were derived following in vitro, in ovo, and/or in vivo laboratory passage (Brugh and Beck, 1992; Brugh and Perdue, 1991; Brugh and Perdue, 1993; Beck et al., 1995; Horimoto
and Kawaoka, 1995; Ohuchi et al., 1989; Perdue et al., 1995; Swayne. et al., 1995). However, no systematic examination has been accomplished to determine if all NP/MP HS and H7 AIVs have equal potential for emergence of HP AIVs.
The acquisition of high virulence for NP and MP HS and H7 AIVs is most consistently related to molecular changes at the cleavage site of the hemagglutinin surface glycoprotein (Kawaoka and Webster, 1988). First, AIVs of low virulence have a maximum of two basic amino acids within the cleavage region. By contrast, AIVs of high virulence have a minimum of four basic amino acids out of five residues in the HA1 subunit immediately upstream from the cleavage site. The increase in basic amino acids at the cleavage site, either as substitutions or insertions, correlated with cleavability of hemagglutinin in trypsin-free CEF cultures and with expression of high lethality in chickens (Bosch et al., 1979). Second, the loss of a glycosylation site at amino acid 13, adjacent to the proteolytic cleavage site of the HA molecule allowed enhanced cleavability and increased virulence. This occurred with the shift in virulence during the 1983/84 AI outbreak in Pennsylvania poultry (Kawaoka et al., 1984).
In November 1993, respiratory problems of undetermined etiology were reported in broiler flocks in Mexico (Charon and Ramirez, 1995). In central Mexico in May 1994, HS AIV was isolated from 6-week-old broilers exhibiting respiratory signs and 12-18% mortality (Charon and Ramirez, 1995). These viruses were categorized as NP AIVs based on chicken pathogenicity tests (Charon and Ramirez, 1995; Senne et al., 1995). In November 1994 in Puebla, Mexico, laying hens exhibited depression and torticollis (Salem, 1995) (M.V.Z.E. Rivera-Cruz, personal communication). Mortality rates (1.2-15.6%) in the field and following laboratory pathogenicity tests (J. Pearson and D. Senne, personal communication) were not consistent with HPAI. However, the amino acid sequence of the AIVs hemagglutinin cleavage site were compatible with HPAI (Garcia et al., 1995; Garcia et al., 1996) (J. Pearson and D. Senne, personal communication). In January 1995 in Queretaro, Mexico, H5N2 AIV was diagnosed in a broiler breeder operation with total cessation of egg production in 6 days and presence of gross lesions compatible with HPAI. Pathogenicity testing in chickens identified this virus as highly lethal and the amino acid sequence of AIV hemagglutinin cleavage site was compatible with HPAI. Furthermore, sequence homology of the HAI segment of various HS AIVs indicated that the Mexican AIVs were most closely related to an HS AIV isolated from a ruddy turnstone in Delaware in 1991 (Garcia et al., 1996; Horimoto et al., 1995). However, the origin of the AIV for the Mexican AI outbreak of commercial poultry is unknown. Possibilities include: 1) direct transmission from migratory bird populations, 2) maintenance of AIVs in backyard poultry, and 3) and transmission from a non-migratory wild bird population.
D. Mycoplasmas
Mycoplasmosis in commercially reared chickens is primarily caused by M. gallisepticum (MG) and M. synoviae (MS). The chronic respiratory disease, synovitis and sinusitis caused by these infections present serious economic problems to the poultry industry. Although these diseases do not necessarily account for high mortality, morbidity is high, and the damaging effects of disease are consequent to lowered egg production, retarded growth, poor carcass quality and predisposition to secondary viral and bacterial infections (Hopkins and Yoder, 1982; Springer, et al., 1974; Timms, 1972). Complicated infections can be severe with associated high mortality (Yoder, 1991).
MG and MS share similar epizootiology. Both are respiratory pathogens of chickens and turkeys (Yoder, 1991), although their host range is not limited to these avian species. MG has been isolated from natural outbreaks in pheasants, partridge, peafowl, quail, parrots, ducks, geese, and house finches. Likewise, MS has been isolated from outbreaks in guinea fowl, ducks, geese, pigeons, quail, and partridge (Kleven, 1991). When spread horizontally, they are transmitted by direct contact with infected poultry, via fomites, or by aerosols. Once respiratory epithelium is colonized by virulent strains, clinical signs include tracheal rates, nasal discharge and coughing. Additionally, MS causes infectious synovitis. Vertical transmission occurs when both MG and
MS infects chicken and turkey reproductive tracts with subsequent spread in hatchlings. Reproductive tract infections in laying chicken flocks can cause lowered egg production and economic loss.
For years the National Poultry Improvement Plan (NPIP) and the American Association of Avian Pathologists (AAAP) have recommended erradication of both MG and MS. Substantial progress has been made in elimination of these agents from primary breeding flocks. Strategies to reduce the adverse impact of MG and MS infection in commercial poultry include, 1) surveillance and eradication programs, 2) use of antimicrobials, and 3) vaccination (Yoder, 1991).
Persistent MG and MS reserviors threaten transmission to commercial mycoplasma-free flocks. They include the large multiple-age egg layer operations where MG is controlled by vaccination with live-attenuated vaccines. Although these immunizations may prevent disease manifestations, they do not prevent infection (Yoder, 1991). Live F strain MG vaccine has been used extensively in pullets to reduce egg production losses that otherwise occur when they are moved to multiple-age layer complexes which have endemic MG (Yoder, 1991). However, following experiental infection, the vaccinal MG F strain was pathogenic for turkeys (Yoder, 1991), and has been associated with MG outbreaks in meat and breeder turkeys under field conditions (Ley et al., 1993). Recently, MG strains 6/85 and ts-11 were produced commercially as live vaccines, and it was reported that both vaccines are poorly transmissible from bird to bird and possess little or no virulence for chickens and turkeys (Abd-el-Motelib and Kleven, 1993; Evans and Hafez, 1992; Whithear et al., 1990). It has not been determined if these live vaccines are virulent under field conditions, particularly when birds are exposed to immunosuppressive viral agents. Determining this necessitates a means to identify each vaccine strain.
Small "backyard" multiple species flocks have long been recognized sources of MG and MS. Today, in addition to these flocks, there is an increasing number of ratite species reared in a variety of conditions. Numerous ratite mycoplasma isolates have been made but are incompletely characterized. This source may present an additional threat for the U.S. poulty industry.
House finches with conjunctivitis leading to blindness and mortality have been reported since 1994 in many eastern states. Between June and September 1994, seven accessions from Virginia, North Carolina and Delaware representing 23 birds (21 house finches, 2 blue jays) were studied. Eleven MG isolations were made (Ley et al., in press). Isolates from the first two positive accessions were confirmed as MG by a commercially available PCR-based test kit (FlockChekTm, IDEXX Laboratories, Westbrook, Maine). In addition, immunofluorescence assays identified the isolates as MG and not closely related species such as M. imitans (Bradbury et al., 1993). An additional PCR-based test indicated that these isolates were not the MG vaccinal F strain. The discovery of MG in house finches presents a new facet to the epizootiology of the disease in poultry and possibly susceptible wild bird populations.
The ability to identify and distinguish current and emerging strains of avian Mycoplasma species is critical to our understanding of the epizootiology of the diseases they cause and effectiveness of interventions such as vaccination. This understanding is required to reduce losses resulting from these diseases.
Diagnosis of mycoplasma infections, particularly MG and MS infections, has had a troubling history. Currently there are serological techniques (hemagglutination inhibition, serum plate agglutination and ELISA) available to detect MG. These tests cannot differentiate between vaccines or field strains. Isolation of mycoplasma from infected flocks and its species identification is very time consuming, taking several weeks. The isolation and speciation of the mycoplasma does not give information on its potential source. For instance are the isolates from a live vaccine or wild bird? Rapid and accurate adjuncts to traditional cultural identication are needed.
With the application of molecular genetic technology, new methods have been developed such as DNA fingerprinting (Kleven, et al., 1988; Morrow et al., 1990), hybridization with species and/or strain-specific mycoplasma DNA (teary et al., 1988; Hyman et al., 1989; Krause et al., 1990; Santaha et al., 1987; Zhao and Yamamoto, 1990), and use of ribosomal RNA gene probes (Yogev, 1988). DNA probes have been shown to be highly specific for detection of MG and MS (Khan et al., 1987, 1989; Zhoa and Yamamoto, 1990). Yet the stringent criteria for
hybridization (Huynh et al., 1985; Nauschurtz et al., 1990; Santha et al., 1987), the requirement for an optimal concentration of organisms (Hyman et al., 1989), and the intricacies of the technology, have not simplified or improved routine laboratory diagnosis of mycoplasmal infections.
Newly developed polymerise chain reaction (PCR) technology has been applied to infectious disease diagnosis of many agents. The assays are rapid, sensitive and specific. These assays build on the information provided by the nucleic acid sequence of defined MG and MS genes. Southern blots and RFLP can differentiate between vaccine strains (Lauerman et al., 1995). These assays are not practical for a diagnostic lab. The arbitrary primed-PCR or random amplified polymorphic DNA (RAPD) analysis (Fan et al., 1994), can also differentiate between the three MG live vaccines. The use of DNA probes in conjunction with PCR is more sensitive than other molecular tests. The IDEXX F-Strain PCR probe assay (IDEXX Laboratories, Inc, Westbrook, ME) can specifically identify F strain. The development of probes specific to ts-11 and 6/85 will allow rapid identification of these two vaccines. Uemori et al. (1992) were the first to develop nested PCR assays to detect pathogenic mycoplasmas in humans and animals. In their study, a nested set of primers was used to amplify an intergenic region between the 16 and 23S ribosomal structural genes. The first set of primers were designed to amplify a conserved sequence within the structural genes of 12 mycoplasma species. A second set of primers amplified the intergenic region itself. Later research showed that these same two primer sets could be used to specifically amplify contaminant mycoplasmas in animal cell cultures (Harasawa et al., 1993). Using this technique, the authors observed that along with variations in length, nucleotide sequences of the spacer regions varied among mycoplasma species. They concluded that this might be useful in species identification (Uemori et al., 1992). This PCR technique has been applied to pathogenic avian mycoplasmas.
The RAPD technique amplifies random DNA sequences using short primers in the PCR. The products of amplification are visualized directly in a gel after electrophoretic separation of fragments and staining with ethidium bromide. RAPD analysis is regarded as a time-saving, costeffective method for studies of genetic diversity, genetic relationships, genetic mapping, DNA fingerprinting and population genetics (Van Buren et al., 1994; Vierling and Nguyen, 1992). Fan et al. (in press) have developed RAPD primers capable of distinguishing among 25 MG strains. Using a slightly modified procedure, others confirmed the utility of RAPD analysis for MG strain identification (Ley et al., in press). Specifically, the RAPD technique can distinguish among the reference strain R and the vaccine strains F, 6/85 and ts-11.
In summary, there is a need to apply these PCR assays in routine diagnostics in veterinary diagnostic laboratories to confirm and establish their efficacy and efficiency using clinical specimens.
Results of conventional serological tests such as the serum plate agglutination (SPA) test, the hemagglutination inhibition test, and the enzyme-linked immunosorbent assay (ELISA) have frequently been confounded because of cross reactions between shared MG and MS epitopes (Avakian et al, 1988; Bradbury and Kleven, 1987; Kleven, 1975; Olson et al., 1965; Opitz and Cyr, 1985, Vardaman and Yoder, 1971). Recent studies have demonstrated a genetic and antigenic relationship between MG and MS (Nauschuetz et al., 1990; Yogev et al., 1989), thus confirming the presence of epitopes common to both species and thereby the difficulty in distinguishing MG from MS. Spurious serological reactions resulting from vaccination of poultry with mycoplasma-unrelated bacterins and the presence of antibodies to such agents as Staphylococcus aureus and Streptococcus faecalis have been cited as factors that frequently complicate the interpretation of test results (Avakian et al., 1988). A number of other factors have been implicated as likely sources of erroneous test results e.g. presence of undesirable contaminating media components in mycoplasma test antigens (Kleven, 1975; Opitz and Cry, 1985; Vardaman and Yoder, 1971; Yogev et al., 1988) and the presence of antibodies to serum components in poultry vaccines (Ahmad et al., 1988; Avakian, 1988; Hopkins and Yoder, 1982; Ross et al., 1990; Springer et al., 1974; Timms, 1972).
The consensus of most researchers is that the success of any serodiagnostic test for MG detection will rest on the availability of a reliable antigen (Avakian et al., 1988; Bradbury, 1988; Kleven et al., 1988; Opitz and Cyr, 1985; Talkington et al., 1985). This is a high priority, since serological survellience is the cornerstone of eradication programs.
A monoclonal antibody (MAb M9) identified a MG agglutinating antigen. This antigenic a potential test antigen. There is observed antigenic variability, both between different strains and among variants of a single species (Kleven et al., 1988; Panangala et al., in press, Rosengarten and Wise, 1990; Thomas and Sharp, 1988). Amid this surface variability, there are some antigenic determinants that are conserved among all mycoplasmas of a given species (Bradly et al., 1988; Hwang et al., 1989; Kenny, 1978; Nichols and Kenny, 1984), thus enabling serological characterization of mycoplasmas into serovars (ICSB, 1979; Khanna et al., 1989; USDA, 1985). One antigen detected in MG is highly conserved in all strains (Morsy et al., 1991; Panangala et al., in press). To date, isolates from different geographic locations in the U.S. react with Mab M9 in Western blot procedures suggesting the agglutinating antigen's conservation among all MG strains.
A wide array of antigenic mycoplasmal proteins have been successfully cloned and expressed as recombinant proteins (Riethman et al., 1987; Stevens and Krause, 1990; Strasser et al., 1991; Su et al., 1988; Taylor et al., 1984). One troubling aspect of recombinant mycoplasma protein expression is that mycoplasma species have a nonstandard genetic code; tryptophan is specified by the codon UGA , the stop codon in all other organisms (Dybvig, 1990).
Despite the tryptophan codon problem, a number of researchers have successfully cloned and expressed mycoplasmal genes (Geary et al., 1988; Khan et al., 1987; 1989; Zhao and Yamamoto, 1989). In a recent study, a genomic fragment coding for the amphiphilic surface lipoprotein p65 of M. hyopneumoniae was successfully cloned and expressed in E. coli (Kim et al., 1990). A 19 kDa recombinant protein corresponding to the carboxy-terminal region of p65 was recognized by both monoclonal and monospecific polyclonal antibody to the gel-purified p65. Serum from swine, obtained after experimental M hyopneumoniae infection preferentially recognized the native amphiphilic p65 lipoprotein and also bound to the recombinant product (Kim et al., 1990). Similar studies with MG have revealed the presence of specific antigens cloned and expressed in E. coli that were recognized by serum from MG -infected chickens (Krause et al., 1990). Precise analysis of many antigen-coding genes that signal events in mycoplasmal pathogenesis have been made possible with innovative molecular biologic procedures (Feldmann et al., 1992; Jacobs et al., 1988; 1990; Lai et al., 1990).
Recently a two candidates for test antigens have been studied. The putative MG cytadhesin (attachment) gene, mgcl , has been cloned and sequenced (Dohms et al., 1992; Keeler et al., 1995). This gene shows 29% amino acid (aa) homology with the major P1 cytadhesin of the human respiratory pathogen M. pneumoniae. In addition, the two genes flanking mgcl have been sequenced. The upstream gene, mgc2 shows 41.6% amino acid homology to M. pneumoniae cytadhesin p30 (Hnatow et al., 1995). Sequence of the gene downstream from mgcl is near completion, and it shows 27% as homology with M. pneumoniae ORFS, an cytadhesin-accessory protein encoded in the P 1 cytadhesin operon. Portions of MGC 1 have been expressed as peptides. Specific polyclonal antibodies have been prepared against MGC 1 and MGC2 using peptide immunogens. Using these antibodies, MGC 1 was found to be trypsin sensitive and surface exposed on the MG cell.
E. Infectious bursal disease virus (IBDV)
Infectious bursal disease (IBD) has been observed in chickens since 1957 (Cosgrove, 1962). This immunosuppressive disease of young chickens is enzootic in many areas of the U.S. (Faragher, 1972; Winterfield, 1969). Chicks infected during the first week of life are permanently immunosuppressed, are more susceptible to pathogenic microbial agents (Fadly et al., 1976; Giambrone et al., 1977; Lucio and Hitchner, 1980; Rosenberger and Gelb, 1978) and do not respond adequately to vaccinations (Allan et al, 1972; Faragher et al., 1974; Giambrone et al., 1977; Winterfield et al., 1978).
The bursa of Fabricius (BF) is the primary target organ of IBDV. IBDV replicates in the immature bursa-derived lymphocytes (B-lymphocytes) of the chicken (Hirai and Calnek, 1979;
Ivanyi, 1975; Yamaguchi et al., 1981). Birds infected with IBDV at one-day of age are completely devoid of serum immunoglobulin G (IgG) and produce only a monomeiic IgM (Ivanyi and Morris, 1976). A permanent decrease in the number of peripheral blood B cells was observed following IBDV infection (Hirai et al., 1979). IBDV has been observed in association with T-lymphocytes (Kauier and Weiss, 1980, Sivanandan and Maheswaran, 1980, Winterfield et al., 1972) but its effect on these cells has not been elucidated. Studies on turkey poults indicated that IBDV infects cells in the BF epithelium at the apex of the plica (Nusbaum et al., 1988). The virus was not observed in cells within the BF follicle. This study suggests that in the turkey, IBDV infects epithelial cells and not lymphocytes; which is consistent with the lack of immunosuppression observed in turkeys (Jackwood et al., 1982; Jackwoad et al., 1984). The nonenveloped IBDV virion displays icosahedral symmetry and is approximately 58-60 nm in diameter (Becht, 1981). The genome of IBDV consists of two segments of double-stranded RNA (MacDonald, 1980, Muller et al., 1979; Steger et al., 1980). Molecular cloning and in vitro translation studies (Hudson et al., 1986) have shown that each RNA segment encodes a single mRNA. The larger mRNA codes for a polyprotein which is cleaved following translation into the maior structural proteins of the virus. The smaller mRNA encodes an RNA polymerise.
Birnaviruses have at least four structural proteins. The designation and molecular weights of the structural proteins observed in IBDV are: VPI (90,000), VP2 (41,000), VP3 (35,000), and VP4 (28,000) (Becht, 1981; Dobos, 1979; Dobos et al., 1979). Additional proteins have been identified (Becht, 1981; Dobos, 1979; Nick, et al. 1976; Todd and McNulty, 1979). It was suggested that they have a precursor-product relationship with the four structural proteins observed in infectious vi.rions (Muller and Becht, 1982). Molecular studies on the largest RNA segment support this hypothesis (Hudson et al., 1986).
Initially IBDV was thought to be a disease only of chickens. However a second serotype of IBDV, isolated from turkeys, (Jackwood et al., 1982; Jackwood et al., 1984; Lukert et al., 1981, McFerran et al., 1980; McNulty et al., 1979) was determined to be a different serotype. Chicken-derived IBDV were designated serotype 1 and the turkey-derived IBDV were termed serotype 2. The serotypes were designated based on antigenic differences observed in virusneutalization and cross-challenge tests (Jackwood et al., 1982). At least one shared antigen exists among serotype 1 and 2 IBDVs viruses and can be demonstrated by immunofluorescence. Both serotypes are present in commercially-reared chickens but only serotype 1 viruses cause disease (Ismail et al., 1988; Jackwood et al., 1982; Jackwood and Saif, 1983; Jackwood et al., 1984; Jackwood et al., 1985). Studies have shown antigenic subtypes (classical and variant) exist within serotype 1 IBDV (Ismail et al., 1990; Jackwood and Saif, 1987; Rosenberger and Cloud, 1985; 1986; Rosenberger et al., 1987; Saif, 1984;1987). Vaccination of chicks with one of these subtypes does not insure birds will be protected from infection and disease from another serotype 1 subtype (Rosenberger and Cloud, 1985; Saif, 1984). Ismail and Saif (1991) demonstrated that vaccination with one serotype 1 subtype did not always protect chickens from challenge with another serotype 1 subtype particularly if a vaccine dose, containing low virus titers, was used. The some vaccination programs to protect against IBD may be due to differences between antigenic subtypes of serotype 1 IBDV (Ismail and Saif, 1991; Jackwood and Saif, 1987; McFerran et al., 1980; Saif et al., 1987).
The distribution of serotype 1 IBDV strains IM and 2512 in chickens has been described (Kaufer and Weiss, 1980; Sivanandan and Maheswaran, 1980; Winterfield et al., 1972). The BF and spleen contained the highest quantities of virus as determined by titration on the chorioallantoic membrane of embryonated eggs (Winterfield et al., 1972). Other tissues which contained virus were the blood, liver, kidney, lung, and thymus. The CU-1 IBDV strain (serotype 1) was at its highest titer in the chicken bursa and spleen at 2 days post-inoculation (Kauser and Weiss, 1980). This virus also spread to the thymus, and liver. Six days following inoculation, IBDV could not be detected in these tissues using a virus plaque assay.
Passage of IBDV in chick-embryo-fibroblast (CEF) cells changed both the biologic properties and structural proteins of the virus (Muller et al., 1986). When virus was passaged at a high multiplicity of infection (MOI), a small plaque variant was observed and at a low MOI, a large plaque variant was observed. The small and large plaque variant populations were stable after the ninth passage in CEF cells. The small plaque morphology remained stable even when the virus was further passaged at a low MOI. Muskett et al. (1985) also reported on the emergence of small plaque variants. Recently, Saijo et al. (1990) plaque purified the small and large plaque variants and observed stable populations through 10 passages in CEF cells. Large et al. (1987) also observed stable large and small plaque morphologies. They reported differences in structural proteins between a population of viruses grown in bursa tissue compared to the small and large plaque viruses grown in CEF cells. It was speculated that cellular factors such as proteases were important in the maturation of IBDV particles and differences in the micro-environment among different host cells could account for the development of defective virus particles. The antigenicity of the small and large plaque viruses was reported to be similar (Saijo et a1.,1990). These studies were conducted using a VN assay. The pathogenicity and immunogenicity of two antigenic variant IBDV strains was studied following 30-40 passages in BGM-70 cells (Tsai and Saif, 1992). Although both viruses lost pathogenicity, their immunogenicity was not altered. Since no attempt was made to separate large and small plaque viruses both plaque sizes were present in the population of virus used for these vaccination /challenge studies. Saijo et al. (1990) reported that both plaque sizes retained immunogenicity in chickens but the large plaque virus had a higher potency compared to the small plaque virus. The large and small plaque viruses were less pathogenic for chicks compared to the wild type virus grown in bursa tissue (Saijo et al., 1990) and the small plaque virus was more attenuated than the large plaque virus (Lang et al., 1987; Saijo et al., 1990).
Detection of IBDV antibodies has been accomplished using a number of different assays. ELISA is often used because it is economical and can accomodate large numbers of samples. Many improvements have been made to ELISA first described by Marquardt et al. (1980). Case et al. (1983) optomized the test using purified antigen. Another modification of the IBDV ELISA used a linear regression equation to predict the ELISA titer of a serum sample from a single dilution (Briggs et al., 1986; Snyder et al., 1986; Snyder et al., 1984). Thayer et al. (1987) reported a correlation between serum ELISA antibody titers and the virus-neutralization (VN) assay for IBDV. Since the development of commercially available ELISA kits for IBDV antibodies, little improvement of this technology has been reported.
Commercially available IBDV ELISA kits detect antibodies to both serotypes l and 2 (Ismail and Saif, 1990). Identification of antibodies to different antigenic subtypes of IBDV is currently only possible using the VN assay (Jackwood and Saif, 1987; McFerran et al., 1980). ELISA systems employ whole virus particles as antigen. One approach to the development of a more specific assay includes expression the IBDV structural protein VP2 for use as an ELISA antigen. Oppling et al. (1991) reported that VP2 contains at least three independent epitopes. Using monoclonal antibodies, Whetzel and lackwood (1995) showed that differences in neutralizing epitopes on VP2 exist between variant and classic viruses. These results suggest that it may be possible to express IBDV epitopes which will only bind antibodies to specific subtypes of IBDV. Several expression systems have been utilized to produce IBDV proteins. Azad et al. (1987) expressed VP2 in Escherichia coli. A recombinant fowlpox virus expressing VP2 protected birds challenged with IBDV against mortality, but not against damage to the bursa (Bayliss et al., 1991). Vaccination of specific-pathogen-free (SPF) hens with a VP2 fusion protein produced in yeast provided passive (maternal antibodies) protection to IBDV challenged progeny (Fahey et al., 1991). IBDV proteins have also been expressed using the baculovirus system (Oppling et al., 1991; Vakharia et al., 1993). Proteins expressed in baculovirus have been produced in amounts of 1 to 500 mg/ml (Luckow and Summers, 1988a; Luckow and Summers, 1988b; Niikura et al., 1991; Smith et al., 1983). There are no reports of using these expression products as antigen in an ELISA.
Objectives
-
Develop and evaluate rapid diagnostic capabilities for the identification of emerging Infectious bronchitis (IBV), infectious larygotracheitis virus (ILTV), mycoplasmas, and infectious bursal disease virus (IBDV).
-
Characterize immuno-suppressive viruses (IBDV) and assess the interaction of underlying immunosuppressive agents (IBVDV and CAV) on emerging respiratory agents.
-
Delineate the epizootiology of emerging mycoplasma and the viruses that cause infectious bronchitis, avian influenza, and infectious laryngotracheitis.
-
Design and implement novel immune and genetic prophylactic strategies for effective control of respiratory diseases caused by emerging IBV, ILTV, mycoplasmas, and IBDV and CAV.
-
Methods
Objective 1. Develop and evaluate rapid diagnostic capabilities for the identification of emerging IBV, ILTV, mycoplasmas, and IBDV.A. Infectious bronchitis virus (MV)
Delaware, Georgia and Connecticut will cooperate in the development and evaluation of direct automated sequencing for identifying field isolates. Delaware, Georgia, and Connecticut will design primers that anneal to conserved sequences flanking hypervariable regions in the S 1 gene. Primer sequences will be evaluated for their ability to anneal all IB V serotypes. For the purposes of designing improved primers, Delaware and Georgia will share unpublished IBV Sl sequence data for the Delaware and California variant serotypes. The primers ability to amplify an unknown (blind) panel of diverse IBV serotypes will be determined. The PCR products will be directly sequenced using a sequences to verify the identity of the IBV serotype. This method for identifying IBV serotypes will be an improvement over exisiting approaches because a new or previously unrecognized serotype can be immediately identified and compared to recognized serotypes. '
Connecticut will construct an IBV genomic cDNA library as a basis for development of group and strain-specific probes and RT-PCR techniques. IBV strains Massachusetts (Mass 41), Connecticut (Cone 46), Arkansas (Ark 99), and Delaware variant (072) will be initially grown in chicken embryo kidney cells (CEK), plaque-purified and characterized by oligonucleotide fingerprinting. The viruses will then be grown in 10-to-1l-day-old chicken embryos, genomic viral RNA isolated by standard procedures, copied into cDNA by reverse transcription and cloned into the plasmid pUC 18 (Sambrook et al., 1989). The screening and identification of MV specific segments of the cDNA will be done by cross-hybridization (DNA:RNA) against a panel of IB V field, and variant strains, and other avian pathogenic viruses of Newcastle disease, avian influenza, infectious laryngotracheitis, reovirus, fowlpox and infectious bursal disease. Clones containing unique sequences will be selected as strain-specific probes. Sequence analysis of the cDNA fragments will be performed (Sambrook et al., 1989) in order to identify DNA primers specific for strains of IBV. Efficacy of the cDNA probes as well as RT-PCR's will be tested for their sensitivities and specificities in experimental infections. Eight-week-old SPF chickens will be inoculated intratracheally with IBV strains, Mass, Conn, Ark, and Delaware variant. Fecal swabs will be taken for 8 weeks to monitor IBV infection using nucleic acid hybridization (labeled cDNA probes) and by standard virus isolation procedures (Lukert, 1980). Blood samples will be taken at the same intervals for serologic monitoring using HI, VN, and ELISA (King, 1988; Lukert, 1980). Isolates of IB V from various geographic locations of the U. S. will also be provided by Delaware
and Georgia. After a successful identification of cDNA probes and RT-PCR primers, additional IBV isolates from field outbreaks and their suspected source of infection will be studied on a comparative basis.
Georgia will improve the RT PCR RFLP test by designing better primers. The procedures for the rapid serotype identification of IBV using RT PCR RFLP have been published (Andresen et al., 1991; Jackwood et al., 1992; Kwon et al. 1993). Georgia and Delaware will attempt to streamline RT PCR procedures by modifying the IBV RNA extraction and PCR methods so that samples taken from the field can be examined directly without the necessity for embryo passage.
Delaware will characterize IBV antigenic determinants. This project will identify serotype-specific peptides for diagnostic purposes. Peptides common to several IBV serotypes will be evaluated as novel vaccines (see Objective 4). A random hexapeptide library constructed in the phagemid, fd-tet (Parmley and Smith, 1988; Scott and Smith, 1990), will be used as a source for the IBV epitopes. This peptide library is unique, such that the hexapeptides are expressed as fusion proteins with the minor coat protein, pIII on the surface of the filamentous phage, fd. The recombinant phage library was amplified in the host Escherichia coli strain, K91Kan . The titer of the genomic library was 3.7 x 10 ltransductants/ml. To elucidate the epitopes of IBV, various strain-specific chicken polyclonal antisera will be used (at least 2 antisera/IBV strain). Through the process termed "panning", phage which bind to the antibodies and therefore, express specific IBVspecific peptides will be separated from unbound phage. The bound phage will then be eluted, amplified in K91 Kan host cells and subjected to two more rounds of panning. After the third round of panning, phage will be mixed with K91 Kan host cells and then plated at low density on medium containing tetracycline to allow growth of transductants. Phagemid DNA from these recombinant bacterial colonies will then be prepared for sequencing. It is expected that at least 50 recombinant clones per antiserum will be sequenced for determination of the consensus peptide sequences (epitopes), as described previously (Cwirla et al., 1990). Phage which bind to IBVspecific antibodies and contain unique peptide sequences will then be tested for their ability to inhibit virus neutralization in homologous assays. The specificity of peptides will also be tested in heterologous neutralization assays.
B. Infectious laryngotracheitis virus
Delaware has identified and sequenced a number of ILTV genes. Several of these genes are structural glycoproteins (gB, gC, gD, gE, gI, gH, gX, gp60, gp67). Efforts will continue to characterize the proteins encoded by these genes. The development of specific immunologic reagents will be used to characterize ILTV glycoprotein maturation and processing. These reagents will also be used to develop specific ELISA assays to monitor ILTV infections in the field.
In addition, a genetically-tagged, live attenuated vaccine strain of ILTV will be constructed by deleting a non-essential glycoprotein gene. Without the gene, birds infected with the mutant virus will not develop antibodies to the corresponding protein. This property can be used to subsequently differentiate the vaccine virus from a field isolate. Methods for constructing mutant strains of ILTV have been developed and published (Guo et al., 1994; Okamura et al., 1994; Schnitzlein et al., 1995). The ILTV gX gene will be targeted for disruption. The gX gene is nonessential and is not involved in the pathogenicity of other herpesviruses. Consequently, its removal should not effect the efficacy of a vaccine strain. The gene will be disrupted by deleting a portion of the coding sequence and replacing it with a gpt-lacZ gene cassette. The resulting plasmid will be co-transfected with ILTV viral DNA into a chicken liver cell line and recombinants will be identified by their ability to make beta-galactosidase and their lack of gX antigen.
C. Mycoplasma
Georgia will develop specific probes for differentiating live MG vaccines from field strains of MG. The probes will consist of a regions complementary to specific sequence within the intergenic region of the individual MG vaccines. The intergenic region between the 16 and 23S ribosomal RNA genes will be sequenced and differences reflecting intraspecies heterogeneity will be used to develop DNA probes that will allow rapid MG identification and differentiation. Intergenic regions of the DNA will be amplified using the PCR technique of Uemori et al. (1992) using the first set of primers (F1 and R1). The purified PCR product will be used in a second amplification using the nested primers. These products will be sequenced and the resulting data analyzed. Subsequent sequencing will be performed using internal primers designed from previously determined sequence. A search for restriction sites within the intergenic regions will be performed. Probes will be designed using results of the sequencing, labeled and used to visualize specific MG strains.
Alabama will develop a simple and specific test for MG diagnosis by cloning and expressing the agglutinating antigen gene. The gene will be identified and characterized. This will be accomplished by constructing an MG (strain PG31) DNA expression library in lambda gtl l and screening it with monoclonal antibodies (Snyder et al., 1987). Delaware will provide Alabama with an lambda gtl l MG S6 genomic library. The libraries will be screened with MAb M9 which recognizes the MG agglutinating antigens) by standard methods (Sambrook et al., 1989). The nucleotide sequence of the identified gene will be determined and the predicted amino acid sequence compared with amino acid sequences of other proteins available in the GenPept and Swiss-Prot databases. This information will be useful in suggesting the function of the agglutinating antigen. The nonstandard genetic code of mycoplasma species (Dybvig, 1990), poses a potential problem for screening expression libraries in E. coli. To minimize the effect of UGA tryptophan codons, screening of the expression library will be conducted in host E. coli strain Y1090-Su7UGA. A hybrid gene will be constucted to produce high levels of the entire or portions of the MG agglutinating antigen recognized by the MAb M9. The entire agglutinating antigen and the fragment encoding the M9 epitope will be separately cloned and expressed in an E. coli expression plasmid. Lysates of cells expressing the recombinant agglutinating antigens will be confirmed by Western blot using the MAb M9. Following purification, the MG agglutinating antigen will be utilized in the development of an ELISA and serum plate latex agglutination (SPLA) assay for routine laboratory MG diagnosis.
The SPLA procedure, previously designed for use with recombinant envelope polypeptide for detection of antibodies to HIV (Quinn et al., 1988), will be modified. The specificity, sensitivity, and stability of the assay reagents will be assessed. Efficacy of ELISA and SPLA assays will use sera specific for different mycoplasma (MG PG 31, MGR strain, MS WVU 1$53, M. gallinarum Ala 22 isolate, M. gallinaceum Ala 6 , M. iowae strain 695) Serum from mycoplasma infected or suspect flocks will be obtained through the Alabama State Veterinary Diagnostic Laboratory. Tracheal swabs taken randomly from the corresponding flocks will be cultured and examined for the presence of mycoplasmas and select isolates will be speciated.
Alabama (Veterinary Diagnostic Laboratory) will obtain unidentified mycoplasma isolates from various diagnostic laboratories throughout the U.S., including Alabama (Dept. of Pathobiology), Georgia, North Carolina and Connecticut. The isolates will be cloned three times to assure purity. Each isolate will be initially tested with avian species-specific PCR assays (Boyle, et al., 1995; Kempf, et al., 1993; Lauerman et al., 1993, LHL and VLvS unpublished primers). Isolates that remain unidentified will be evaluated using PCR-restriction fragment length polymorphism (RFLP) analysis and grouped (Lauerman et al., 1995). The 16S rRNA gene will be sequenced from a representative in each group and the sequence information used to select speciesspecific PCR primers using sequence alignment. Other standard diagnostic techniques, such as biochemical tests (Golf, 1994), immunoassays (Goll, 1994; Imada et al., 1987), and immunoglobulin receptors (Lauerman and Reynolds-Vaughn, 1991, Lauerman et al., 1993) will be
utilized for further characterization. General mycoplasma PCR primers will be used to generate amplicon (DNA amplification product) from the down stream end of the 16S rRNA gene through the spacer region to the upstream end of the 23S rRNA gene of unidentified isolates. The PCR amplicons will be reacted with four restriction enzymes (MseI, Tsp509I, DraI, and RsaI), and the electrophoretic patterns of RFLP evaluated (Lauerman, et al., 1995). Amplified DNA fragments will be isolated and the 16S rRNA sequence determined. For fragments longer than 500-600 bp, additional primers, derived from internal sequence will be used to complete the entire fragment. (Weisburg et al., 1989). Many mycoplasmal 16S rRNA sequences have been determined and provide the basis for a systematic phylogenetic analysis of these microorganisms (Weisburg et al., 1989). Species-specific PCR primers will be selected from 16S rRNA gene sequence information using computer alignment studies (Lauerman et al., 1993; LHL and VLvS, unpublished data). The 16S rRNA gene sequence information on various mycoplasmas will be obtained. The suitability of selected PCR primers will be determined by computerized sequence alignment studies. In addition, PCR assays with stock cultures of known avian mycoplasmas will be conducted.
The pathogenicity of selected mycoplasma isolates will be assessed in one-day-old SPF chickens inoculated by the footpad or ocular routes. Chickens will be observed for clinical signs, euthanatized and necropsied at 7, 14, and 28 days postinoculation. Specimens will be collected for histopathology, mycoplasma culture, PCR assays, and serological evaluation.
North Carolina will develop a database for avian Mycoplasma species and strain identification by RAPD analysis. The RAPD technique described by Fan et al. (Avian Dis., In press), will be modified as required to optimize all performance parameters, especially reproducibility. The ability of an optimized RAPD to distinguish among MG strains will be validated using known reference and vaccine strains. MG isolates from poultry and other birds e.g. house finches made by our laboratory and acquired from collaborators will be analyzed by RAPD. RAPD patterns will be photographed and the images digitized using a scanner, or digital images may be captured directly using a digital camera. A computer-assisted gel analysis system such as Dendron (Solltech Inc., Oakdale, IA) will be used to analyze RAPDs and build a database of MG strain identities. Briefly, the gel analysis system will correct, process and analyze RAPD gels in order to compare banding patterns. Similarity coefficients are computed between banding patterns and a dendrogram is generated. Results will be stored in a database which provides the capacity to retrospectively compare the fingerprint of every new strain with any or all strains previously analyzed.
An attempt will be made to finalize and standardize RAPD methodology and select a gel analysis system with input from collaborators at Georgia. Ideally, a uniform standard might emerge that could be widely utilized and whose data could be shared regionally, nationally and internationally via the Internet.
Initially, these efforts will focus on identification of MG vaccine strains and the molecular epidemiology of the MG outbreak in house finches. However, this capability will be readily expanded 'to impact on other aspects of MG disease, and then extended to other pathogenic avian Mycoplasma species (MS, M. meleagridis, M. iowae). North Carolina will exchange avian mycoplasma isolates with Alabama for the purposes of developing a database for avian Mycoplasma species and strain RAPD identification.
E. Infectious bursal disease virus (IBDV)
It is important to define the effects of host systems on antigenic configuration since this could have an enormous impact on any diagnostic reagents prepared from virus propagated in a fashion that would preferentially select for particular epitopes or other in vitro or in vivo characteristics. Delaware will assess the effects of host systems) (bursa of Fabricius, embryo or cell culture) on the virulence and antigenic configuration of several standard- and variant-type infectious bursal disease viruses. Infectious bursal disease virus propagated in the bursa of Fabricius and adapted to grow in embryos and/or chicken embryo fibroblasts will be monitored for
changes in relative pathogenicity or shifts in antigenicity as evaluated with monoclonal and polyclonal antibodies. Currently-available vaccine strains and field isolates will be compared to one another and, in the case of the vaccine strains, to the "parent" virus when possible.
Ohio will concentrate on the molecular characterization and comparison of IBDV serotypes. IBDV strains will be cloned and the VP2 gene sequenced (Jackwood et al., 1989; Kibenge et al., 1990) to develop rapid diagnostic assays. These tests will employ specific cDNA probes in a dot blot hybridization test (Henderson and Jack-wood, 1990; Jackwood, 1990; Jackwood et al., 1989; Jackwood et al. 1990), a reverse transcriptase polymerase chain reaction -restriction endonuclease (RT/PCR-RE) assay (Jackwood and Jackwood, 1994) (Henderson and Jack-wood, 1990; Jackwood, 1990; Jackwood et al., 1989; Jack-wood et al. 1990). The VP2 protein from the Del-A strain IBDV will be expressed in the baculovirus expression system (Henderson et al., 1992) for the purposes of developing a highly strain-specific enzyme-linked immunosorbent assay, the OSU-ELISA. Recombinant viruses expressing the VP2 protein from the Del-A strain of IBDV will be tested for specific neutralizing epitopes using defined monoclonal antibodies. The OSU-ELISA differs from commercial ELISA kits because it iws strain specific. Alabama (Veterinary Diagnostic Lab) will cooperate with Ohio in the evaluation of molecular diagnostic assays.
Objective 2. Characterize immunosuppressive viruses (IBDV) and assess the interaction of underlying immunosuppressive agents (IBDV and CAV) on emerging respiratory agents.
Ohio will import highly virulent strains of EBDV from The Netherlands, Turkey, and Taiwan. These strains have caused major epidemics in the old world in the last seven years but have not been detected in the U.S. The strains will be adapted to tissue culture and used as inactivated preparations to produce antibodies in chickens. Ohio's USDA importation permit limits use of live virus to in vitro study. Sera from immunized birds will be used to conduct in vitro cross neutralization studies. Chickens will immunized with the inactivated EBDV strains and will be challenged with American strains (classic and variant) to study the cross-protective characteristics of these viruses. Results from the above studies should provide a rational basis for prevention of these infections should they appear in the U.S. at some later time.
Delaware will evaluate the impact of virulent, intermediate and avirulent IBDVs on susceptibility to chicken anemia virus. Once defined, chickens that are potentially compromised immunologically by the dual infections) will be exposed to different pathotypes of infectious bronchitis virus (IBV) to assess the impact of relative immunosuppression on the emergence of increased susceptibility to IBV in vaccinated and unvaccinated birds. Results from these assessments will better define the role of common immunosuppressive agents, including IBDV vaccines, on the emergence of respiratory pathogens such as IBV.
Delaware will assess the impact of IBDV infections on the immune responses to two putative cytadhesins, MGC l and MGC2, found surface exposed on MG (Dohms et al., 1992, Keeler et al., 1996). These proteins will be evaluated as vaccines in Objective 4. Inoculation of susceptible SPF chickens with EBDV given at hatching and three-weeks of age will use the variant A strain IBDV At 4,6, and 8 weeks of age IBDV-infected and IBDV-noninfected controls will be inoculated with MGC1 and MGC2, recombinant peptides. At weekly intervals for 6-weeks post inoculation, the chickens will be bled and Harderian glands removed. The onset of local (GH extracts) and systemic antibody production to MGC1 and MGC2 will be evaluated using immune precipitation and immunoblotting procedures. At 6-weeks post immunization, remaining birds will be challenged with MG S6 strain via the air sac route. At 10-days post challenge, all birds will be bled and sampled for tracheal swabbings to detect MG. The incidence and severity of lesions will be evaluated.
Objective 3. Delineate epizootiology of emerging mycoplasma and the viruses that cause infectious bronchitis, avian influenza, and infectious laryngotracheitis
A. Infectious bronchitis virus (IBV)
Georgia and Delaware will continue to monitor the various serotypes and variant viruses involved in outbreaks of the disease. The S-1 gene of "new" serotypes will be sequenced. Georgia and Delaware will examine the pathogenicity of new IBV isolates.
New York will study the pathogenesis of IBV infection in chickens to elucidate the mechanisms of intracellular virus persistence and cellular injury. IBV persistence and shedding following acute IBV infection has a definite impact on the epizootiology of the disease. The goals of this research are: 1) to identify cells which are infected by I13V during acute and persistent phases of infection, 2) to compare the cellular tropism of virulent and avirulent IBV strains, and 3) to identify the forms) in which IBV exists intracellularly during acute and persistent phases of infection. Chickens. viruses and sampling schedule: Groups of 2-week-old chickens will be intranasally inoculated with IBV strains Delaware variant (DE-072) and Mass 41. Tissues (trachea, lung, cecal tonsil, bursae of Fabricius, and spleen) will be collected at post-inoculation days 3, 7, 10 and 14, and subsequently at weekly intervals until chickens are one-year old. Oviduct tissues will be collected only at certain specified intervals. Virus isolation. IBV antieen and genome detection. Virus isolation attempts will be performed in embryonated eggs using routine procedures (Gelb, 1989). IBV antigens will be detected using Immunochistochemical studies. These studies along with virus isolation data will provide a comprehensive picture of IBV tissue isolation data as well as tissue tropism, differences in the tissue tropism of different IBV strains and duration of persistence of IBV in the various tissues and cells. IBV genome will be detected by in situ hybridization (Poulet et al. 1995) using a DNA probe synthesized from IBV nucleocapsid (N) gene earlier cloned in plasmid pBT 327. Since IBV N gene sequences are highly conserved, we expect this probe to hybridize to 072 RNA as it is known to hybridize to M41 RNA. If this probe is found unsuitable for the detection of 072, we will use the 072 sequence data available from Delaware and synthesize a complementary oligo probe. Alternatively, in situ PCR will be performed if tissues from IBV infected chickens are found to be negative for IBV RNA by in situ hybridization. It is possible that IBV RNA may exist in quantities that are below the detection limits of the procedure.
B. Avian influenza virus (AIV)
USDA ARS, Ohio, and Delaware will collaborate to study the ecologic interrelationships of avian influenza viruses. Ohio and Delaware will provide fecal or cloacal swab samples of migratory waterfowl and shore birds (Delaware only) from the Midwest and Atlantic flyways to USDA ARS. Standard virologic procedures will be used for AIV isolation and characterization (Beard, 1989). All HS and H7 isolates will have HA1 segment of the hemagglutinin gene sequenced and compared with standard poultry and wild bird isolates maintained at the Southeast Poultry Research Laboratory. Methodology for sequencing and analysis will use published procedures (Garcia et al., 1996; Perdue et al., 1995). Relationships between different isolates will be determined and reported.
The potential of avirulent AIVs to mutate and become highly virulent will be assessed. All HS and H7 AIV isolates will be tested in a model system that favors emergence of highly pathogenic derivatives (Beck et al., 1995; Brugh and Beck, 1992). Sequence differences between mildly and highly pathogenic AIVs will be compared to determine the sites) of change responsible for virulence shift and determine adjacent common sequences that favor virulence the molecular changes associated with high virulence.
C. Mycoplasmas .
Alabama will conduct a retrospective study among poultry processing plants to determine rates of condemnation for airsacculitis. Results of the retrospective study will be used to develop a sampling frame for a prospective investigation of the involvement of mycoplasmas in airsacculitis condemnation. Monthly samples of respiratory tracts from birds with airsacculitis will be collected from a random sample of processing plants that are stratified by state. A systematic random sample of tissues will be collected from each plant. The samples will be based on the monthly condemnation for airsacculitis determined retrospectively, and 5% of the condemnation samples will be targeted for further evaluation. Veterinary diagnostic laboratories in the U.S., will be relied upon to supply unidentified avian mycoplasma isolates with flock histories to aid in epidemiology studies. Mycoplasma isolates will be obtained from Alabama and Georgia.
North Carolina will utilize the RAPD analysis to assess the epizootiology of emerging mycoplasmas. Georgia will use MG strain specific probes to assess the epizootiology of emerging mycoplasmas.
Objective 4. Design and implement novel immune and genetic prophylactic strategies for effective control of respiratory diseases caused by emerging IBV, ILTV, mycoplasmas, and IBDV.
A. Infectious bronchitis virus (IBV)
Texas will examine the CTL responses to various U.S. strains of IBV in order to identify T-lymphocyte cross-reactive epitopes associated with the S 1 and N proteins. Cross reactive epitopes from the S 1 or the N proteins will be evaluated for their cross-protective potential against heterologous IBV strains. Heterogeneity of IBV strains will be determined by infection of chicks using a CTL assay with target cell expressing various strains of IBV or IBV proteins. Groups of 1-week-old chickens will be infected with one of various strains of IBV by intranasal intraocular routes (Collisson et al., 1990). They will be monitored for respiratory signs of infection. Spleens will be collected from four infected animals four to eight weeks later. Sera will also be collected and the presence of MV specific antibody will be determined using ELISA and western blots (Purr and Collisson, 1993). Effector cells will be collected from spleens following Ficoll-paque gradient separation of single cell suspensions. Target cells generated from a syngeneic CK cell line, infected and labelled with 51Cr. The spleens of age-matched uninfected chickens will serve as controls.
The CTL assay will be done with target cells labelled by overnight incubation with 51 C r (MacCubbin & Schierman, 1986). Background release will be determined. MHC restricted activity will be determined by using targets from heterologous (non-matched) birds. Specificity will be determined using heterologous viruses. In designing recombinant vaccines, it is necessary to choose viral proteins, if not epitopes, that will elicit maximum immunity. Texas will concentrate on the IBV S1 and the N proteins which have been cloned, sequenced and expressed in recombinant vectors. The SFV vector carrying S1 or N genes of Arkansas or other IBV strains will be used to transduce CK cells (Wang et al., 1994). Expression of the IBV proteins will be determined by IFA In addition, the CTL response of chickens to the N and S 1 genes will be determined. Chicks will be inoculated at 1-day of age using a gene gun supplied by Agracetus with plasmid DNA containing the N and S1 gene for which sequences are available. IBV-specific CTL and serum antibody responses will be examined prior to and after IBV challenge. IBVspecific CTL responses to homologous and heterologous serotypes will be evaluated in order to identify viral epitopes with cross-protective potential. Delaware will collaborate with Texas in performing challenge studies to evaluate the immunogenicity cross-reactive epitopes.
Georgia will clone the S1 and S2 glycoprotein genes of Ark IBV into the Baculovirus
expression vector using standard materials and procedures (Miller, 1988; O'Reilly et al., 1990; Yoden et al., 1989). Briefly, the IBV cDNA will be cloned into a transfer plasmid which has an insertion site flanked by sequences homologous to the polyhedrin gene promoter region of the Autographa californica nuclear polyhedrosis virus (AcMNPV), a Baculovirus used as an expression vector. The IBV gene will be cloned into the AcMNPV expression vector by cotransfecting an insect cell line (SF9 cells) with both the transfer plasmid carrying the IBV gene cDNA and the AcMNPV. Recombinant viruses will be detected in the insect cell culture due to the production of a visually selectable plaque phenotype which occurs when the polyhedrin gene of the AcMNPV is substituted with a foreign gene. Screening recombinant baculovirus vectors. Several recombinant expression vectors will be selected and evaluated for production of the IBV glycoproteins. Cells infected with a recombinant expression vector will be assayed with S 1 and S2 specific monoclonal antibodies (Mab) using Western blotting. In addition, the infected SF9 cells expressing recombinant IBV S1 and S2 glycoproteins will be examined by electron microscopy to determine if spikes are being formed. If the nascent S glycoprotein is not cleaved into S 1 and S2 subunits by the host cell enzymes, similar to reports in the literature for bovine coronavirus spike protein (Yoo et al., 1991), we will use site directed mutagenesis (Oste, 1988; Sambrook et al., 1989) to modify the proteolytic cleavage site so that SF9 cell enzymes will cleave the nascent S glycoprotein. Baculovirus expressed glycoprotein immunogenici studies. Expression vectors which successfully produce the IBV S glycoproteins will be evaluated for antigenicity and immunogenicity in a pilot study involving SPF chickens. Chickens will be inoculated with the recombinant protein using several routes. Serum from these chickens will be tested using a commercially available enzyme-linked, immunosorbant assay (ELISA) to measure antibody specific for IBV. The recombinant glycoproteins will also be adsorbed to 96-well microtiter plates and used in an ELISA against chicken sera known to have positive titers to IB V. These tests will give a measure of antigenicity of the recombinant proteins. To evaluate the immunogenicity of the recombinant proteins, the vaccinated chickens will be given a challenge dose of virulent IBV by the intratracheal route. Five days later, some of the chickens will be sacrificed and their tracheas examined by histopathological examination for evidence of damage due to IBV. Tracheal tissue will be processed for isolation of IBV. The chickens will be examined for evidence of clinical signs of IBV infection. Delaware will cooperate with Georgia in the evaluation of promising IBV vaccines.
Georgia will evaluate nucleic acid (DNA) vaccines for IBV. The Sl gene from Ark IBV will be cloned into the pBC12/CMV/IL-2 expression plasmid (Dr. B.R. Cullen, Duke University)(Cullen, -1986). That plasmid containing the H7 gene of influenza virus under the control of the CMV immediate early promoter was used by other researchers to immunize chickens against lethal influenza challenge (Fynan et a1.,1993). Standard cloning procedures will be used (Gubler and Hoffman, 1983). The human IL-2 cDNA sequence will be removed from the pBCI 2/CMV/IL-2 plasmid by digestion with the HindIII and BamHI restriction enzymes. The IB V S 1 gene will be obtained from the plasmid pMJTAS 1 Ark (created in our laboratory) by digestion with EcoRV and BamHI then ligated into the pBC12/CMV vector (with the IL-2 sequences removed) to create plasmid pMJCMV/S1Ark. The S1 gene will be under control of the CMV immediate early promoter. Expression of the S 1 gene will be verified in vitro by transfecting several different cell lines with pMJCMV/S1 Ark using the Lipofectin reagent (Life Technologies, Gathersburg, MD) according to the manufacturers recommendations. The glycoprotein will be visualized by indirect immunofluorescence (IIF) using monoclonal antibodies or polyclonal antisera specific for the Arkansas serotype of IBV. Nucleic acid (DNA) vaccine efficacy studies. In vivo immunogenicity studies in SPF chickens will be conducted. At one-day of age chicks will be vaccinated with approximately 30 ug of plasmid DNA intramuscularly and at 14-days of age the birds will be bled then vaccinated intramuscularly with 100 ug of plasmid DNA Half of the birds in each group will be necropsied at four weeks of age. The remaining birds will be challenged with pathogenic IBV (Ark DPI strain). The birds will be observed daily and necropsied at seven days post-challenge. At necropsy, clinical signs and lesions will be recorded, tracheal swabs will be collected for virus isolation/detection using a PCR-based DNA probe diagnostic test. Various tissues including
trachea, kidney, liver, and spleen, will be fixed in formalin for histopathology. Sera will be collected and tested for antibodies to IBV by ELISA. If protective immunity is not induced in the birds we are prepared to clone the S2 glycoprotein gene into the pBC12/CMV/IL-2 plasmid and use it in conjunction with the pMJCMV/S1 Ark plasmid in immunogenicity studies. Delaware will cooperate with Georgia in the in vivo evaluation of promising E BV vaccines.
Georgia will evaluate a eukaryotic subunit vaccine for IBV. A novel system for synthesizing the S1 and S2 glycoproteins of IBV will be utilized in an attempt to create an efficacious subunit vaccine. The eukaryotic transient-expression system described by Fuerst et al. (1986) utilizes recombinant vaccinia virus expressing the bacteriophage T7 RNA polymerise. When that virus is co-transfected into a continuous cell line along with plasmid DNA containing the S 1 and S2 genes behind a T7 promoter, the 77 polymerise synthesizes the S 1 and S2 mRNA and the cell machinery translates those genes into protein. Vaccinia virus VTF7-3 (ATCC 21 53-VR) was obtained from the American Type Culture Collection (Rockville, MD). The S 1 and S2 genes from the Ark DPI strain of IIBV will be obtained from plasmids pMJTAS1Ark and pMJTAS2Ark respectively, and cloned using standard procedures into the pET-17b plasmid (Novagen, Madison, Wl) which contains the T7 promoter and terminator necessary for transcription of the genes into mRNA. The genes will be cloned into separate plasmids and they will be cloned into a single plasmid as the native spike gene containing the S1 and S2 subunits with the proteolytic cleavage sequence between the two subunits. Once the genes are cloned and verified by sequencing (Singer et al., 1977) they will be co-transfected into cells using the Lipofectin reagent (GibcoBRL, Gaithersburg, MD) according to the manufacturers recommendations along with the VTF7-3 vaccinia virus. Several different cell lines will be used to express the S 1 and S2 glycoproteins including vero cells and HeLa cells. The expressed proteins will be analyzed by IFA using Mab and polyclonal antibodies. Eukaryotic subunit vaccine immunogenicity studies. A pilot study will be conducted in two-week old SPF chickens to determine the immunogenicity of the expressed S1 and S2 proteins as described above. Delaware will cooperate with Georgia in the evaluation of promising IB V vaccines.
Delaware will determine the feasibility of using anti-sense oligonucleotides as a way of
inhibiting IBV replication by targeting essential viral genes and non-coding regions that are
common to all serotypes. Ultimately, the information generated could be used to develop
genetically IB V-resistant commercial chickens when the technology to produce transgenic poultry
is available. Antisense oligonucleotides will be used to inhibit two major transcriptional events in
the replication of the Beaudette strain of IBV. The first transcriptional event that will be targeted is
the RNA polymerase binding site at the 3' non-coding end of the IBV genome. Interference with
the binding of the enzyme to the binding site by anti-sense oligos should inhibit the synthesis of the
negative strand template and dramatically reduce replication at this critical early event. IBV
conserved sequences at the non-coding region at the 5' end (+ strand) and 3' end (- complement)
of the MV Beaudette genome (NIH GenBank accession M95169) (Boursnell et al., 1987) have
been identified using cluster analysis (Higgins and Sharp, 1988) (DNAStar, Inc., Version 1.03,
1993). An oligo complementary to the RNA polymerise binding site will be used to pre-treat Vero
cells 2-4 hr prior to inoculation with the Beaudette strain of IBV. "Nonsense" oligos of the same
length and composition will be included as controls. To achieve optimal specificity, we will
initially use standard phosphodiester anti-oligos but these may be degraded by nucleases.
liposomes. Various concentrations of anti-oligos will be incubated with Vero cells 2-4 hr prior to
the addition of IBV at a moi of 0.1 and 1.0. Growth curves will be performed and infectivity titers
will be determined (Cunningham et al., 1972). Total RNA (Favaloro et al., 1980) will be
determined for infected aand control cultures. The second area of the replication cycle of IBV to be
targeted is the homology regions at the initiation sites of the the A (nucleocansid). B. C
(membranes D E (spike). and F (RNA polymerase ) genes in order to prevent the synthesis of m-
RNAs. Conseved sequences at the initiation sites for leader primed trancription for m-RNAs A
(nucleocapsid), B, C (membrane), D, E (spike), and F (RNA polymerise) have been identified.
An anti-oligonucleotide to each of the six m-RNAs will be used alone and in various combinations
in Vero cells subsequently infected with the Beaudette strain of IBV. Oligos will be 18 mers designed to bind to the core homology region and to the four complementary nucleotides on each side of the homology region. Growth curves and mRNA synthesis will be analyzed as described above. The effect of oligos on the growth of heterologous IBV serotypes will be evaluated to assess the potential applicability of anti-oligo MV strategies for poultry. Anti-oligos that inhibit the Beaudette strain, a Massachusetts serotype, will be tested against heterologous,IBV serotypes, Arkansas and Connecticut. Unlike Beaudette, most IBV strains do not grow in Vero cells. Therefore, chicken kidney cells (CKC) or chicken embryo fibroblast (CEF) cell cultures with trypsin added to the culture media (Otsuki and Tsubokura, 1981) will be used. CEFs were suitable for demonstrating the antiviral effects of anti-oligos on the replication of Rous sarcoma virus (Zamecnik and Stephenson, 1978). CEF and CEK are routinely prepared in our lab using standard procedures (Gelb et al., 1987a;1987b). Growth curves using Arkansas and Connecticut will be performed to determine viral titers in cells treated with promising anti-oligos. Viral RNA levels will be assessed as described.
B. Infectious laryngotracheitis virus (ILTV)
Delaware and Illinois have developed considerable experience in constructing and testing recombinant strains of fowl pox virus expressing the ILTV glycoprotein B gene. Such a recombinant fowl pox virus has been shown to protect birds from a virulent ILTV challenge (Keeler and Poulsen, 1995). Delaware and Illinois will collaborate on constructing and testing strains of recombinant fowl pox expressing additional or multiple ILTV antigens. Likely gene candidates for insertion into fowl pox include the gD, gC and gH genes.
Successful completion of Objective 1 will be the first step in developing ILTV into a recombinant vaccine vector. Once a selectable foreign gene, like the lacZ gene, has been successfully inserted into a nonessential ILTV gene, such as gX, the next phase is to insert a gene encoding a protective antigen for another avian pathogen. Recombinant vaccines expressing the fusion (F) and/or hemagglutinin-neura.minidase (HN) genes of Newcastle disease virus (NDV) have been successfully constructed and tested using either fowl pox virus or herpesvirus of turkeys as the viral vector (Boursnell et al., 1990a; Boursnell et al., 1990b; Morgan et al., 1992). Neither of these vector systems induces adequate local immunity in the chicken respiratory tract. ILTV, being a respiratory pathogen, should be examined as an alternate vector system for use with avian respiratory pathogens. Delaware will use the techniques described above to clone the NDV F and HN genes into the gX gene of ILTV. Recombinant viruses wilt be evaluated for their ability to protect birds from challenge by ILTV and local or systemic challenges by NDV using established methods (Hastings, 1991; Morgan et al., 1992).
C. Mycoplasmas
Delaware will evaluate the immunogenicity and protective effects of MGC 1 and MGC2 recombinant peptides following challenge with virulent MG S6 strain. The MGC 1 peptide contains a 177 as recombinant protein expressed in the pQE30 vector (Qiagen, Inc., Chattsworth, CA). The region expressed is exposed on the cell surface and contains no trptophan,TGA codons. MGC2, a 304 as protein, was expressed as a fusion protein in pMALc following repair of a TGA codon by site-directed mutagenesis. Experiments will involve single entity (MGCl or MGC2 peptides alone), and MGC l and MGC2 combined as a single vaccine. Challenges will be after one to three immunizations using virulent MG S6 via the airsac route of inoculation. Controls will include non immunized chickens and chickens immunized with commercially available inactivated MG bacterin. The immune antibody response to both MGC1 and MGC2 will be monotored using immunoprecipitation and immunoblotting assays.
E. Infectious bursal disease virus (IBDV)
Delaware will cooperate with Maryland in the development of a subunit vaccine for CAV using recombinant techniques. The genomic ssDNA will be cloned and the complete nucleotide sequence determined. The VP1 and VP2 genes will be subcloned and expressed in a model baculovirus expression system. The expressed proteins will be characterized with available monoclonal antibodies and then evaluated as immunogens serologically and via challenge. If successful the proposed research would allow for the production of a safe CAV vaccine for use in breeding stock to protect progeny thereby minimizing immunosuppression associated with this agent and providing for increased resistance against respiratory pathogens.
Ohio will cooperate with Illinois to express IBDV genes in avian pox virus for the purposes of developing a recombinant vaccine.
Measurement of Progress and Results
Outputs
- Molecular tests for identifying/diagnosing isolates of infectious bronchitis virus (IBV).
- Identification of gene sequences necessary for the development of ELISA tests for infectious laryngotracheitis virus (ILTV).
- Preliminary work on developing ILTV vaccines.
- Development of genetic probes for differentiating wild strains of Mycoplasma from live vaccine strains.
- Development of simpler molecular tests for detecting Mycoplasma strains in poultry populations.
- Development of a database for avian Mycoplama species and strain identification by RAPD analysis.
- Development of tests for identifying strains of infectious bursal disease virus (IBDV) and avian influenza virus (AIV).
- Continued work on development of prophylactic strategies for poultry respiratory diseases.
Outcomes or Projected Impacts
- Diminished losses from poultry respiratory diseases.
- Increased profits for the poultry industry from reduced losses from respiratory diseases.
- Reduces hazards for consumers from safer poultry products.