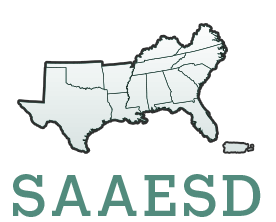
S1063: Quantification of best management practice effectiveness for water quality protection at the watershed scale
(Multistate Research Coordinating Committee and Information Exchange Group)
Status: Inactive/Terminating
S1063: Quantification of best management practice effectiveness for water quality protection at the watershed scale
Duration: 10/01/2014 to 09/30/2019
Administrative Advisor(s):
NIFA Reps:
Non-Technical Summary
Statement of Issues and Justification
Despite years of dedicated implementation effort and study, it remains difficult to give definite answers to such questions as “Are pollution control measures like best management practices (BMPs) really improving our environment, and, if so, by how much?; and How and where do we get the best environmental return per BMP investment/implementation made?” To answer these seemingly simple, straightforward questions requires complex tools and study methods some of which have yet to be developed. Numerous studies have investigated the costs of watershed management activities needed to meet water quality goals. A good example is the Chesapeake Bay Watershed TMDL, which was completed in 2010 and which calls for annual reductions in nitrogen, phosphorus, and sediment of 25, 24, and 20%, respectively. Estimated costs of the required watershed improvement activities in the state of Virginia alone (one of 6 states in the watershed) are estimated to be $13.6 to $15.7 billion by 2025 (Senate Finance Committee, 2011). Ninety percent of this funding is targeted for rural and urban watershed protection activities that target diffuse pollution sources (agriculture, urban stormwater, and on-site wastewater disposal). The remaining 10% of the cost involves wastewater treatment system upgrades. A significant portion of the required diffuse pollutant (nonpoint source NPS) reductions will be achieved with physical and managerial best management practices (BMPs), which studies have shown can be the most cost effective means of reducing pollutant loads. For example, estimated costs in dollars per pound of annual nitrogen reduction are >$200/lb for stormwater retrofits, and $15.80 to $47.40/lb for waste water treatment plant upgrades. Contrast that with $4.70/lb for cover crops, $3.20/lb for conservation tillage, $3.20 /lb grassed buffers, and $1.50/lb for constructed wetlands (Jones et al., 2011). If the costs of the Chesapeake Bay Program are extrapolated across the United States to other legally mandated water quality improvement programs (Great Lakes, Mississippi River Basin, Everglades, San Francisco Bay, Albemarle-Pamlico Sound, etc.) the cost is potentially in excess of $100 billion/year for the foreseeable future. A major challenge with current models used for watershed management planning is that these models, in general, poorly simulate BMP performance. Consequently there is considerable uncertainty in model predictions and investments in water quality improvement activities almost always fail to achieve projected benefits. Currently, simulations of BMPs are limited by the inability of the models to realistically simulate the spatial placement of BMPs and the lack of the ability to account for pollutant accumulation with in the BMP and/or declining pollutant removal efficiencies of the BMP over time. Further, many models cannot account for variable BMP performance as a function of the intensity and duration of the pollution-producing precipitation events. Typically, BMP effectiveness is included in models by applying a reduction or pass-thru factor that represent the pollutant removal potential of a given BMP or suite of BMPs. These pollutant reduction or pass-thru factors do not maintain the pollutant mass balance and do not account for the potential release of previously trapped pollutants with time. Additionally, BMP effectiveness is often based on the ratio of output to input pollutant concentrations, thus, research that uses very large input concentrations will observe better efficiencies than are perhaps representative of most realistic conditions (Dittrich et al., 2003). In an ideal world, one would execute a suite of controlled experiments at the field- and watershed-scales to directly measure BMP implementation effectiveness. This is clearly not practical for many reasons, not the least of which is a lack of “control” landscapes. Thus, there is a need to find ways to use the “real world” landscapes as living experiments to evaluate BMP effectiveness. Paired watershed studies offer a proven method for evaluating the cumulative effects of BMPs, particularly under varying hydro-climatic conditions (Bishop et al., 2005), but it is not necessarily easy or entirely feasible to see which BMPs are the most effective with this kind of approach. Similarly, long-term monitoring may provide insights into system-wide changes in a watershed, but it may be difficult to associate these changes with specific BMPs or combinations of BMPs, and trends may be difficult to interpret in light of the impact of exogenous variables such as weather variability or climate change. Many studies that have purported to demonstrate the cumulative effectiveness of BMPs are almost entirely based on model results (e.g., Sorrano et al., 1996; Santhi et al., 2003) and thus are subject to problems with incomplete understanding of the relevant fate-transport mechanisms involved. This is especially true when models that require calibration of BMP-associated pollutant export coefficients are used. Using models to assess BMP performance can also be problematic since some models are not capable of simulating processes or management practices at the scale at which most BMPs are implemented (Garen and Moore, 2005). While the principal focus of the project proposed here is to evaluate the effectiveness of BMPs at the watershed scale, BMP effectiveness at the sub-watershed scale is also important. For example, the interest of individual farmers, land developers, and others may be more focused on what constituents/pollutants are leaving their land as opposed to the impacts of those pollutants downstream. Consequently, this project will also investigate techniques for estimating BMP effectiveness at the subwatershed/field scale. This project postulates that the best way to gain insights into the effectiveness of water quality management practices is to combine sub- and watershed-scale water quality monitoring with distributed hydrological water quality modeling. In order to accurately simulate BMP performance, models must be based on physical pollutant fate and transport processes. The models must also be parsimonious to minimize problems of equifinality that may arise. In fact, model calibration should be minimized to the extent possible. The models developed through the work proposed here should also be spatially-distributed to a fine enough resolution to capture the impacts of individual BMPs (Garen and Moore, 2005). Ideally, these distributed model should be validated, i.e., tested, against both distributed and watershed-scale (stream) observations. This multistate project will develop and evaluate models that can be used to predict the effectiveness of BMPs and BMP implementation at the farm, subwatershed and watershed scales, improve and assess the ability of watershed management models to address emerging environmental issues, and develop methods to quantify modeling and monitoring uncertainty as affected by model representations of watershed processes and model input data. The overall aim of the project is to develop tools and techniques that can be used to accurately predict BMP performance effectiveness across a range of spatial scales. Achieving this aim will permit more informed and cost effective watershed management decision making. A multistate effort is required because of the plethora of BMPs that must be investigated, and because of the site-specific nature of selected landuses and associated BMPs. Successfully completion of this project will reduce the uncertainty and costs associated with BMP implementation efforts and improve local, regional, and national water quality.
Objectives
-
Monitor water quality from a variety of watersheds with a range of conditions (e.g., differing landuse and associated implemented BMPs, varying geographic/geologic conditions),
-
Develop and evaluate models for predicting BMP performance and water quality at the field and watershed-scales when considering climate change, and
-
Develop methods to quantify modeling and monitoring uncertainty as affected by model representations of watershed processes and model input data.
Procedures and Activities
Objective 1: Monitor water quality from a variety of watersheds with a range of conditions (e.g., differing landuse and associated implemented BMPs, varying geographic/geologic conditions) After decades of research, questions still remain about the ability of BMPs to mitigate NPS pollution, and the processes at play that determine /define BMP pollution mitigation performance. Ever increasing demands for pollution mitigation are increasing the need for effective and wide-spread of BMP implementation. To efficiently achieve this increased level of implementation, new insights into the pollutant fate and transport processes at play in various BMPs and the role of various forcing-functions on BMP performance are needed. To gain these insights we will collect data at the BMP, field and watershed scale. Collected data will include, but not be limited to flow and water quality (e.g. concentration data of sediment, nutrients, and pathogens), pollutant removal kinetics, various climactic variables, etc. Data collection will also likely involve measuring fluxes at the soil-air, water-air, and soil-aquifer interfaces to better understand BMP performance. BMPs that will be targeted for this research include: riparian buffer zones/filter strips, constructed wetlands, stream-side fencing, sediment detention practices, nutrient management, integrated pest management, denitrifying bioreactors, conservation tillage, infiltration practices, porous pavement, green roofs, grass waterways/vegetated swales, soil carbon enhancement, etc. An example of a specific type of activity that will be undertaken to address Objective 1 involves monitoring manufactured treatment devices (MTDs) that are typically are used to control sediment and nutrient losses in urban areas. Examples of MTDs include: rainwater harvesting systems, bioretention systems, sediment separation devices, and floating treatment wetlands. The MTDs use a variety of technologies to achieve potentially greater treatment efficiency while reducing space requirements, which is advantageous in urban settings. Verifying the performance of MTDs is difficult however because runoff water quality is highly variable and manufacturers often do not have appropriate testing facilities to provide performance data required for informed BMP selection. There is also a lack of standardized protocols for field testing of MTDs and presentation of performance data. In order to address these issues, Virginia initiated the Virginia Technology Assessment Protocol (VTAP) to assess the performance of urban stormwater BMPs and VTAP assessment of newly developed MTDs is now required by the Commonwealth of Virginia to verify that MTDs meet performance goals (Sample et al. 2012). The state of Washington has recently developed a similar program, the Washington State Dept. of Ecology Technology Assessment Protocol-Ecology (TAPE). These programs fill a critical missing gap in providing independent and impartial evaluations of BMP effectiveness for nutrient and sediment removal. Project participants from Virginia will share their expertise in the development of standardized protocols for BMP performance and the project participants will collaboratively develop and implement new testing protocols to better characterize the performance of new and existing BMPs. Results obtained from this objective will feed an existing knowledge base of BMP efficiencies and will further provide the necessary data, which will then be used in developing improved BMP evaluation methodologies and water quality management. Objective 2: Develop and evaluate models for predicting BMP performance and water quality at the field and watershed-scales when considering climate change. Execution of this objective will involve reviewing the latest modeling methods, and assessing existing advantages and shortfalls associated with these methods. It will also involve developing new or adapting existing models or submodels to describe BMP-specific pollutant mitigation fate and transport processes. The focus will be on developing BMP submodels that are capable of being integrated into existing field- and watershed-scale models when possible. The development of new more spatially explicit models will also be investigated. BMP submodels to address urban, agricultural, and silvicultural NPS pollution will be pursued. An example of a model-specific activity that will be undertaken to address this objective involves modifying the Soil and Water Assessment Tool (SWAT) watershed scale model (Arnold et al., 1998; Neitsch et al., 2011) to more accurately represent landscape transport processes. The current hydrologic response unit configuration will be re-coded to account for overland transport between upland landscape units to better simulate the spatial placement and effects of BMPs and land use. Additional Climate change (e.g. changing precipitation and temperature regimes and increased precipitation and temperature variability) may have significant impact on the generation/loss of various water quality pollutants (e.g. sediment, nutrient, pesticide, pathogens) (Parajuli, 2010; Godfray et al., 2010). We propose to use existing watershed models like SWAT and the Hydrological Simulation Program FORTRAN (HSPF; Duda et al. 2012), to assess the impact of climate change on pollutant generation and BMP performance. In addition, we may develop new/modify existing watershed scale models to be able to assess BMP performance and the field and subwatershed scale. Future climate change scenarios will be developed based on global or regional climate model projections of precipitation, temperature, and CO2. Observed daily precipitation and temperature data for the long-time period will be used in the climate models such as the LARS-WG a stochastic weather generator model to downscale future climate data (Rackso et al., 1991); or reports based on Inter-governmental Panel on Climate Change (IPCC) reports (IPCC, 2009). Further, currently available other downscaled climate data can also be utilized such as ensemble multi-model multi-scenario and multi-projection climate data from the World Climate Research Programmes (WCRPs) Coupled Model Inter-comparison Project Phase 5 (CMIP5). Any new model development under taken in Objective 2 will be driven both by data availability and by the desired model application (i.e., what kinds of questions will any models be taksed to inform). Models developed under Objective 2 will be calibrated and validated for streamflow and other parameters using available observed data such as the United States Geological Survey (USGS). Model performance will be evaluated using commonly used metrics such as mean, median, coefficient of determination (R2), and Nash-Sutcliffe Efficiency index (NSE) (Moriasi et al., 2007; Parajuli 2009). Objective 3: Develop methods to quantify modeling and monitoring uncertainty as affected by model representations of watershed processes and model input data. While the results of the first two project objectives will advance our knowledge about the potential impacts of BMP implementation at the watershed scale, still more analysis is required to be able to answer questions like “How confident are you that the BMPs being implemented in our watershed will really improve downstream water quality, and by how much?” Ideally, to provide informed answers to this seemingly simple question one would have access to the apportionment of uncertainties at the social, economic, modeling and monitoring levels, as they all interact together (e.g. Chen et al., 2007). In this objective, we propose to analyze uncertainties associated with the models which we will use/develop to predict BMPs impacts at the watershed and subwatershed scale. We will conduct uncertainty analyses on model inputs and outputs to produce a complete picture of the confidence that can be associated with a given watershed model/BMP performance prediction. In addition, we will explore how to best communicate the results of these types of uncertainty analyses to stakeholders and decision makers such that the information is presented in a form usable to these varied audiences. To accomplish this objective, it is crucial that the uncertainties at each level in the watershed/BMP simulation/analysis process be addressed sufficiently well so as to develop an integrated uncertainty assessment. We propose to use uncertainty analysis methods developed by Campolongo et al. (2007) and Ruano et al. (2012) to apportion the relative importance of each modeling variable/data input in the overall uncertainty and will derive associated modeling, monitoring, and reporting guidelines and recommendations. Timeline Regional project meeting to agree upon priority BMPs, emerging environmental issues, and uncertainty analysis goals for research and identify research teams to address each objective and/or sub-objectives. (1st quarter of 1st year of project) Conduct literature reviews on identified priority BMPs, and emerging environmental and uncertainty issues for submodel/methods development and testing. (2nd quarter of 1st year of project) Identify short and long-term sources of BMP performance and hydrologic data that can be used for BMP assessment and submodel/monitoring development and uncertainty analysis. (3rd quarter of 1st year of project) Develop collaborative proposals to fund priority BMP, submodel/monitoring development, and uncertainty analysis research. (Year two and thereafter) Develop improved protocols and methods for incorporating uncertainty analysis into watershed and subwatershed scale models. (Year two and thereafter) Incorporate and validate developed BMP submodels and uncertainty analysis methods into watershed and subwatershed scale models currently being used for watershed management planning. (Year two and thereafter) Annual project meeting to discuss project progress, additional research needs, new collaborations, and plans for coming year. (Annually) Conference with published proceedings detailing the results and findings of the project. Conference would be public with relevant presentations and papers from project participants and others. (5th year)
Expected Outcomes and Impacts
- Coordination of research and outreach programs involving the use of BMPs for watershed management and mitigating diffuse pollution and the effects of climate change and other emerging environmental issues.
- New knowledge and exchange of ideas/information/data on the biophysical functioning of BMPs to enable the development of better BMP submodels and watershed and subwatershed models for simulating BMP effectiveness and uncertainties associated with their performance.
- Publication of joint research articles on BMP performance and monitoring and modeling methods at the watershed and subwatershed scales.
- Evaluation and standardization of uncertainty analysis techniques for quantifying uncertainties associated with BMP performance and model predictions of BMP effectiveness at the watershed and subwatershed scales.
- A conference on quantification of best management practice effectiveness for water quality protection at the watershed and subwatershed scales.
Projected Participation
View Appendix E: ParticipationEducational Plan
The results of this project will be disseminated through the project web page, publications of participants, annual CRIS reports, and the concluding project conference on quantification of best management practice effectiveness for water quality protection at the watershed scale.
Organization/Governance
The recommended Standard Governance for multistate research activities include the election of a Chair, a Chair-elect, and a Secretary. All officers are to be elected for at least two-year terms to provide continuity. Administrative guidance will be provided by an assigned Administrative Advisor and a CSREES Representative.
Literature Cited
Arnold, J. G., R. Srinivasan, R. S. Muttiah, and J. R. Williams. 1998. Large-area Hydrologic Modeling and Assessment: Part 1. Model Development. J. American Water Resources Association, 34(1): 73-89.
Bishop, P.L., M.R. Rafferty, and J.L. Lojpersberger. 2006. Effectiveness of whole farm planning and implementation in achieving water quality improvement and protection of New York City water supplies: Final Report to the Watershed Agricultural Council. New York State Dep. of Environmental Conservation, Albany, NY. 35 p.
Campologo, F., J. Cariboni and A. Saltelli. 2007. An effective screening design for sensitivity analysis of large models. Environ. Modell. Softw. 22: 1509-1518.
Dittrich, T.M., L.D. Geohring, M.T. Walter, and T.S. Steenhuis. 2003. Revisiting buffer strip design standards for removing dissolved and particulate phosphorus. p. 527534. In Total maximum daily load environmental regulations II conference proceedings, 812 Nov. 2003, Albuquerque, NM. ASAE Publ. 701P1503. ASAE, St. Joseph, MI.
Garen, D. C., and D. S. Moore. 2005. Curve number hydrology in water quality modeling: Uses, abuses, and future directions. J. Am. Water Resour. Assoc. 41:377-388.
Godfray, H. C. J., J. R. Beddington, I. R. Crute, L. Haddad, D. Lawrence, J. F. Muir, J. Pretty, S. Robinson, S. M. Thomas, and C. Toulmin. 2010. Food Security: The Challenge of Feeding 9 Billion People. Science, 327(5967): 812-818.
IPCC (Inter-governmental panel on climate change). 2009. The Physical Science Basis. Contribution of working group I to the fourth assessment report of the IPCC. Cambridge University Press, Cambridge, United Kingdom and New York, NY: USA. Available at: http://www.ipcc.ch/publications_and_data/ar4/wg1/en/contents.html. Accessed 7 December 2012.
Jones, CY, E Branosky, M Selman, and M Perez. 2010. How Nutrient Trading Could Help Restore the Chesapeake Bay. How Nutrient Trading Could Help Restore the Chesapeake Bay. February 2010 Working Paper, World Resources Institute, Washington, DC. Accessed: 09/05/2013. Available at: http://pdf.wri.org/working_papers/how_nutrient_trading_could_help_restore_the_chesapeake_bay.pdf
Moriasi, D. N., J. G. Arnold, M. W. Van Liew, R. L. Binger, R. D. Harmel, and T. L. Veith. 2007. Model evaluation guidelines for systematic quantification of accuracy in watershed simulations. Trans. ASABE, 50(3): 885-900.
Neitsch, S. L., J. G. Arnold, J. R. Kiniry, and J. R. Williams. 2011. Soil and Water Assessment Tool, Theoretical Documentation. Available at: http://swatmodel.tamu.edu/documentation. Accessed 7 December 2012
Novotny, V. 2003. Water quality: Diff use pollution and watershed management. 2nd ed. John Wiley & Sons, New York, NY.
Parajuli, P. B. 2010. Assessing Sensitivity of Hydrologic Responses to Climate Change from Forested Watershed in Mississippi. Hydrological Processes, 24(26): 3785 3797
Parajuli, P. B., Nathan O. Nelson, Lyle D. Frees, and Kyle R. Mankin. 2009. Comparison of AnnAGNPS and SWAT model simulation results in USDA-CEAP agricultural watersheds in south-central Kansas. Hydrological Processes, 23(5): 748-763.
Racksko, P., Szeidl, L., and Semenov, M., 1991. A serial approach to local stochastic weather models. Ecol. Model. 57 (1-2), 27-41.
Ruano, M. V,, J. Ribes, A. Seco and J. Ferrer. 2012. An improved sampling strategy based on trajectory design for application of the Morris method to systems with many input factors. Environ. Modell. Softw. 37: 103-109.
Sample, D.J., Grizzard, T.J., Sansalone, J., Davis, A.P., Roseen, R.M., Walker, J., 2012. Assessing performance of manufactured treatment devices for the removal of phosphorus from urban stormwater. Journal of Environmental Management, 113, pp. 279-291, doi: 10.1016/j.jenvman.2012.08.039.
Santhi, C., J.G. Arnold, R. Srinivasan, and J.R. Williams. 2003. A modeling approach to evaluate the impacts of water quality management plans implemented in the Big Cypress Creek watershed. Total maximum daily load (TMDL) Environmental Regulations-II, Proc. of the November 2003 Conf. of the ASAE, Albuquerque, NM
Senate Finance Committee. 2011. Chesapeake Bay TMDL Watershed Implementation Plan: What Will it Cost to Meet Virginias Goals? Senate of Virginia. Richmond. Accessed: 09/05/2013. Available at: http://sfc.virginia.gov/pdf/retreat/2011%20Retreat/Presentation_Final%20PDF%20for%20Website/5.Chesapeake%20Bay%20TMDL%20FINAL.pdf
Sorrano, P.A., S.L. Hubler, S.R. Carpenter, and R.C. Lathrop. 1996. Phosphorus loads to surface waters: A simple model to account for spatial pattern of land use. Ecol. Appl. 6(3):865-878
Walter, M.F., T.S. Steenhuis, and D.A. Haith. 1979. Nonpoint source pollution control by soil and water conservation practices. Trans. Of the ASAE 22:834840.
Walter, M.T., V.K. Mehta, A.M. Marrone, J. Boll, P. Gérard-Marchant, T.S. Steenhuis, and M.F. Walter. 2003. Simple estimation of prevalence of Hortonian flow in New York City watersheds. J. Hydrol. Eng. 8:214218.