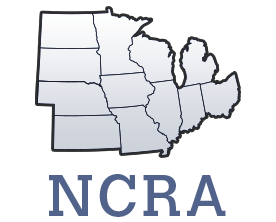
NC_OLD1184: Molecular Mechanisms Regulating Skeletal Muscle Growth and Differentiation
(Multistate Research Project)
Status: Inactive/Terminating
NC_OLD1184: Molecular Mechanisms Regulating Skeletal Muscle Growth and Differentiation
Duration: 10/01/2010 to 09/30/2015
Administrative Advisor(s):
NIFA Reps:
Non-Technical Summary
Statement of Issues and Justification
The overall goal of this cooperative, multi-state, multidisciplinary, basic research project is to increase the efficiency of lean meat production in domestic animals. Meat, derived from skeletal muscle, is one of the most economically important outputs of animal agriculture. Rapid, efficient deposition of lean muscle tissue is essential to economical production of high-quality meat which is critical to both the economic success of producers and the health of consumers. Development of successful strategies to increase efficiency of muscle production requires increased understanding of the biological processes regulating differentiation and growth of muscle in meat animals. Although we have made significant progress toward this goal under the auspices of the current NC-1131 project, continued advances in molecular and cellular biology methods (microarrays, siRNA, gene transfer, real-time RT-PCR, etc) have provided many of the tools necessary to dramatically advance our understanding of these processes. Consequently the goal of this multi-state, multidisciplinary, basic research project is to utilize these tools to elucidate molecular and cellular processes that regulate differentiation and growth of skeletal muscle; thereby, providing the basic knowledge necessary to increase the efficiency of lean meat production in meat-producing animals.
This proposal for renewal of the NC-1131 project describes the collaborative effort of researchers from 18 different Agricultural Experiment Stations to characterize specific aspects of the molecular and cellular mechanisms that regulate skeletal muscle growth. Major points that support the continuation of this important, fundamental research project for the next five years are:
1. The project relates to a major agricultural problem. Meeting consumer needs for a high quality product while maintaining profitability of production, decreasing environmental impacts, and minimizing use of natural resources will require improvements in the efficiency of meat production in domestic animals. These efforts rely directly on fundamental knowledge of the biological mechanisms that regulate muscle growth.
2. The project relates directly to identified national agricultural research priorities: Objective 2.2 of the CSREES Strategic Plan for 2007-2012 is Provide research, education, and extension to increase the efficiency of agricultural production and marketing systems. Specifically, Performance Criteria 2.2.11 Improve understanding of fundamental animal physiological processes (CSREES knowledge area 305) of this Objective is supported by the efforts of NC-1131. Improving the efficiency of meat production in domestic animals, based on fundamental knowledge of the mechanisms of muscle growth, is directly related to this goal.
3. One of the Actionable Strategies of CSREES Objective 2.2 is "Support the recruitment, training, retention, graduation, and placement of the next generation of research scientists, educators and practitioners in the food and agricultural sciences." An important component of this project has been and will continue to be the training of undergraduate and graduate students, and postdoctorates in basic fields that will relate to long-term improvements in agricultural productivity.
4. The crosscutting research areas established by the NCRA Committee in the Agricultural Production, Processing and Distribution area include "Develop improved animal, plant, and microbial production, production and marketing systems that are competitive, profitable and environmentally sound over the long term." This project provides the basic research necessary to achieve this goal.
5. Broadening and enriching the knowledge base about genomics, including the utilization of molecular techniques (gene mapping, est sequencing, functional genomics) is another goal of the NCRA that research conducted in this project will impact. These efforts are consistent with CSREES Objective 2.2, specifically Performance Criteria 2.2.9 Develop and apply information and technology for genetic improvement of animals (CSREES Knowledge Area 303), and Performance Criteria 2.2.10 Map and understand the genome of agriculturally important animal species (CSREES Knowledge Area 304). The project supports current efforts to map genes important in animal production by providing fundamental information about the regulation and expression of genes during muscle differentiation and growth. New cutting-edge methodology, such as microarrays to assay gene expression of thousands of genes simultaneously, and mass spectroscopy for protein and peptide identification, open new avenues for determination of biological mechanisms.
6. The NC-1131 Committee continues to be highly productive. A total of 179 refereed papers, 11 book chapters/Theses and 92 abstracts have resulted from years 2006-2008 of the current five-year NC-1131 project, and additional publications are expected by the September 30, 2010, termination date. Many of these papers are in high quality basic science journals, attesting to the quality of the work and justifying the basic approach of this project. Substantial progress has been made under each of the specific objectives of the current project.
7. The NC-1131 project is both a multi-state and a multidisciplinary project, involving the effort of investigators at 18 different State Agricultural Experiment Stations. The Principal Investigators represent a variety of basic science disciplines that complement each other and provide the expertise necessary to complete the objectives.
8. The project continues to involve a strong cooperative effort between the various units. Cooperative efforts during the current project have involved activities such as exchange of reagents, including well-characterized monoclonal and polyclonal antibodies, cDNA probes, and microarray platforms, sharing of knowledge and techniques, joint use of equipment and techniques available at particular stations, and joint publication of research results. Numerous collaborative projects are described in the procedures for the proposed revision. The committee feels strongly that the collaborations in this project would have been substantially more difficult to establish and maintain outside the framework of a funded regional project.
9. The members of the NC-1131 committee have been highly successful in obtaining outside support to fund the research. Funding from the USDA NRICGP (AFRI) Program, NIH, NSF, health-related granting agencies, and industry sources has been essential to carry out the work and to maintain the high level of productivity of the group, and this record of outside funding is expected to continue.
10. The members of the NC-1131 committee organized and participated in a symposium at the joint meeting of the Animal Science, Dairy Science and Poultry Science Associations in San Antonio, TX (2007). It was entitled Growth and Development - Livestock and Poultry: Transcriptional Factors and Cell Mechanisms for Regulation of Growth and Development.
In summary, this project describes a fundamental research approach to an important agricultural problem. The investigators at the cooperating stations have demonstrated a high level of productivity, and are, thus, capable of making substantial progress towards the objectives outlined in this revised project proposal.
Related, Current and Previous Work
There is no duplication between this project and any other funded multi-state (regional) project. Searching the CRIS Multi-State Projects database using the keywords "muscle and (growth or differentiation or development)" retrieved only a single project - NC-1131. Searching this database with the single keyword "growth" yielded only 3 active projects: NC-1131, and 1 project focusing on warm water aquaculture and 1 project dealing with plants. Thus, both the current and the proposed NC-1131 projects are unique among currently funded multi-state projects. The only related Regional project is NE-148, "Regulation of Nutrient Use in Food Producing Animals," and there is little real overlap, as NE-148 deals primarily with nutrient use and hormonal regulation, does not focus on skeletal muscle, and does not focus on understanding the molecular and cellular mechanisms regulating muscle growth. Funded projects dealing with identification of growth-related genes as part of gene mapping efforts do exist, but these do not focus on regulation. Thus, there is no duplication between this project and other multi-state/regional projects.
Muscle differentiation (myogenesis) and growth are regulated by a number of growth factors (GF). Members of the NC-1131 regional project have made significant contributions to our understanding of the roles of insulin-like growth factors (IGFs) (1-12), fibroblast growth factors (9;13;14), transforming growth factor-beta (TGF-beta) and myostatin (1; 2; 4; 15-22), anabolic steroids (1-4; 7; 23), hepatocyte growth factor (HGF) (24-28), leptin (29-33), Insulin-like growth factor binding proteins (IGFBP) (5; 11; 16; 34-39), ERK (40), E2F (41), and Beta-adrenergic agonists (46;47) in regulating muscle proliferation and differentiation in meat animals. However, much remains to be done. The discovery that muscle growth in cattle is dramatically affected by a deletion mutation in the myostatin gene illustrates the significant impact that even a single growth factor can have on muscle growth (48; 49). Similarly, although anabolic steroid implants have been used commercially for over 40 years to increase rate and efficiency of muscle deposition in feedlot steers (50), only recently has research done cooperatively by NC-1131 project members begun to elucidate their mechanism of action on skeletal muscle growth (1-4; 7; 23). This illustrates the fact that, although the general effects of many GFs on muscle differentiation and growth have been recognized for a number of years, their exact mechanism of action is not known, partially because the effects of specific GFs on muscle are impacted by complex interactions of several factors unique to muscle tissue. For example, the bioactivities of the IGFs in muscle are dramatically affected by nutritional status (51), the level of IGF in muscle tissue (1; 52; 53), presence of IGF receptors (54-56) and interaction with a family of IGF binding proteins (IGFBPs) (1; 37; 38; 57-59). Although growth factors and hormones may reach muscle via the circulatory system, many cell types found in muscle tissue, including myogenic cells and fibroblasts, can synthesize specific GFs including IGFs (1; 53), IGFBPs (16; 37; 60), FGF-6 (61), myostatin (62), TGF-beta (63), and HGF (24). Thus, GFs produced locally in muscle can act to regulate proliferation and differentiation of myogenic cells. For example, mice overexpressing IGF-I in muscle tissue show increased muscle deposition and increased satellite cell numbers as compared to control mice. Additionally, mice overexpressing specific IGFBPs show alterations in muscle mass and growth rate. Furthermore, mice in which myostatin expression in muscle has been knocked out have 30 - 40% greater muscle mass than litter mate controls and double muscled cattle in which myostatin function has been impaired by a naturally occurring mutation in the myostatin gene have significantly increased muscle mass. Additional research is needed to characterize the expression of specific GFs in muscle and to define their endocrine, autocrine, and/or paracrine actions in regulating muscle growth in meat-producing animals.
Growth factors affect muscle through complex intracellular signal transduction pathways that are initiated by binding of growth factors (GFs) or hormones to specific intracellular or cell surface receptors (64-67). The formation of the ligand receptor complex initiates intracellular signal transduction mechanisms resulting in activation of specific molecules and/or expression of specific genes that regulate muscle differentiation and growth. The phosphatidylinositol 3-kinase (PI 3-kinase) pathway has been reported to stimulate differentiation of myogenic cells (65; 66; 68; 69) while the mitogen-activated protein kinase (MAPK) pathway stimulates proliferation at the expense of differentiation (64; 70; 71). Members of the Rho GTPase family have been shown to either stimulate or suppress differentiation depending on identity of the family member (72). Additionally, members of the TGF-beta family, including TGF-beta and myostatin, suppress both proliferation and differentiation of cultured muscle cell lines and primary myogenic cells via the Smad pathway (73-75). Recently, there is increasing evidence that the giant muscle protein titin, which constitutes nearly 10% of the protein in myofibrils, may participate in signal transduction, using mechanical stretch signals to affect gene expression (76). Some titin binding proteins re-distribute from the myofibril to the nucleus to provide signals for hypertrophy. Most of the evidence for this latter phenomenon has been obtained in the heart, but similar mechanisms are likely to also function in skeletal muscle. Research conducted by NC-1131 members has begun to elucidate the mode of action in stretch-induced activation of muscle cells (77-79). There is also evidence that the P94 calpain is tightly bound to titin; this suggests that titin breakdown may be a key initial step in muscle protein turnover and myofibril remodeling that occurs during growth (80). Signal transduction pathways interact with each other (cross-talk) in complex ways that are not completely understood at present. Although the general intracellular pathways utilized by a number of growth factors have recently been identified, little is currently known about how these specific pathways function in muscle to control proliferation and differentiation of embryonic myogenic cells and satellite cells or hypertrophy of muscle fibers in postnatal muscle. Furthermore, although the previous NC-1131 project yielded significant new information on Raf/MEK/MAPK (81-83) and Smads (84), the majority of what is known has been determined in laboratory animals such as mice and rats and may not be directly applicable to meat-producing animals. These reports along with a large number of other reports establish the importance of identifying and characterizing intracellular signal transduction pathways activated by specific growth factors in muscle and elucidating their role in muscle differentiation and growth in meat animals. Consequently, more emphasis will be placed on this research area in the renewal proposal.
Results obtained during the previous NC-1131 project (85-97) as well as the work of other researchers have shown that the extracellular matrix (ECM) surrounding muscle cells may play an important role in muscle growth and differentiation (98; 99). The ECM functions by directly interacting with membrane receptors and by modulating growth factor activities. The activity of growth factors such as Fibroblast growth factor type (FGF)-2, hepatocyte growth factor (HGF), transforming growth factor-beta (TGF-beta) and IGFBP are regulated by binding to proteoglycans (100-102). Cell surface heparan sulfate proteoglycans may modulate terminal myogenesis by acting as low affinity receptors for growth factors such as FGF-2 (103-105) and HGF (106). The fact that expression and cellular localization of proteoglycans is highly regulated during muscle differentiation (107) also suggests that these molecules may play a role in the differentiation process.
Myogenic regulatory factors (MRFs) are basic helix-loop-helix (HLH) transcription factors that initiate myogenesis and regulate the transcription of muscle specific genes (reviewed by (108-110)). There are four members of this family; MyoD1 (111), myogenin (112; 113), myf-5 (114) and MRF4/herculin/myf-6 (115-117). MRFs form heterodimers with ubiquitously expressed E2A, E12 and E47 gene products (118; 119), and these heterodimers bind to the DNA consensus sequence CANNTG, also referred to as an E box. Forced expression of any one of this family of transcription factors (myoD, myf-5, myogenin or MRF-4) in nearly every cell type can force cells to reprogram their chromatin and establish gene expression profiles similar to that of normal skeletal muscle cells (120). In fact, some argue that mechanisms underlying control of these "powerful" transcription factors may be a nodal point for understanding and controlling muscle development. The target of these dimers, the E box motif, is found in the regulatory regions of numerous muscle-specific genes including the MyHC isoform genes (121-125). Skeletal muscle consists of a heterogeneous collection of muscle fibers differing in their ability to contract and metabolize energy (126). Classification of each fiber type in muscle is a rather nebulous process but relies on the functional capabilities of each fiber. Speed of contraction is related to the type of myosin heavy chain (MyHC) isoform contained within a muscle fiber (127), and therefore, changes in the expression of these genes collectively will ultimately impact the muscle fiber functionality. Muscle fibers change to accommodate a myriad of physiological stimuli, such as hormonal changes, innervation state, aging, nutrition, and other physiological parameters. From a practical stand point, the relative proportion of these fibers in a muscle is related to its inherent ability to grow and its susceptibility to disuse atrophy. Although the aforementioned stimuli are well-documented, the molecular mechanisms controlling changes in MyHC gene expression as well as that of other muscle specific genes are not understood.
Transcription factors affecting gene expression in muscle work in concert with several growth factors, as evidenced by the fact that bHLH-mediated up-regulation of muscle specific genes only occurs when growth factor presence and abundance in the environmental milieu is permissible. These growth factors include such well-known proteins as the insulin-like growth factors, the family of fibroblast growth factors, transforming growth factors and many others. In addition, expression of extracellular matrix proteins may play a significant role in regulating differentiation and proliferation of myogenic cells. As discussed earlier in this review, myostatin provides a good illustration of the biological significance and the potential impact of growth factors in the biomedical and agriculture communities. Mutations in the myostatin gene are responsible for the well-known double-muscled syndrome in cattle. Myostatin is a member of the transforming growth factor-beta superfamily and is a negative regulator of skeletal muscle growth (128). Mice and cattle deficient for myostatin by naturally occurring or knock-out mutations of the gene exhibit dramatic increases in skeletal muscle mass (48; 49; 129-131). The condition in several cattle breeds such as Belgian Blue and Piedmontese is associated with mutations in the myostatin gene and results in excessive muscle fiber formation (hyperplasia). Skeletal muscles of these cattle contain almost double the number of fibers compared with other cattle breeds, whereas fiber size is nearly equal or slightly larger than normal animals (132). This tremendous increase in muscle fiber number is associated with increases in muscle mass of > 20% and decreases in fat (132). Although other growth factors likely work in concert to modulate muscle growth, the myostatin "story" serves to illustrate the tremendous gains in muscle growth that are possible by elucidating the mechanisms controlling the expression and action of a specific protein regulating muscle growth (133-135). The recent development of techniques such as microarray analysis and real-time RT-PCR make it feasible to more completely characterize the expression of known molecules such as myostatin and to identify other proteins that may regulate proliferation and differentiation of muscle cells. During the current NC-1131 project, a porcine complementary DNA (cDNA) microarray was produced that contained expressed sequence tags (EST) derived from whole embryo and adult skeletal muscle, and differential display PCR products from fetal and postnatal muscle (136-143). This microarray will be used to identify and characterize specific genes responsible for controlling prenatal myogenesis and postnatal myonuclear accretion.
Accumulation of muscle protein mass depends on the balance between rate of muscle protein synthesis and rate of muscle protein degradation. It is now well-established that muscle protein turnover has an important and sometimes the predominant role in rate of skeletal muscle growth. Decreasing rate of muscle protein turnover also is directly related to increasing the efficiency with which ingested nutrients are converted to muscle tissue. There are several classes of proteins in skeletal muscle; the contractile or myofibrillar proteins constitute over 60% of total muscle protein and are responsible for the contractile properties and many of the functional properties of muscle. Hence, studies on muscle protein turnover need to focus on the myofibrillar proteins. The myofibrillar proteins, however, also present unique challenges to their turnover. After synthesis, the myofibrillar proteins are rapidly assembled into myofibrils, which are tubular-like structures that extend continuously from one end of the muscle cell to the other. The contractile properties of myofibrils require that this continuous structure be maintained during turnover; hence turnover of the myofibrillar proteins must be done without disrupting this structure. Moreover, the myofibril is a dynamic structure that contains a number of proteins, some that are directly responsible for contractions (actin and myosin), others that serve to regulate contractile activity (e.g., troponin, tropomyosin) and others that seem to be involved in maintaining the myofibrillar structure (e.g., alpha-actinin, titin, nebulin). In addition, a number of proteins such as the intermediate filament (IF) proteins, bind to the periphery of the myofibril and are important for linking the contractile proteins to the sarcolemma and maintaining lateral registration of the myofibrils within a muscle cell. The dynamic interactions among these different groups of proteins, and the mechanism used by cells to accomplish their turnover without disrupting myofibrillar function is poorly understood.
Myofibrillar protein turnover, as is turnover of all proteins, is mediated by proteases. There are four proteolytic systems in muscle cells that are present in sufficient quantities to catalyze intracellular protein turnover.
1. The lysosomal system. Proteases in this system, the cathepsins, are located inside lysosomal structures and have acidic pH optima. The primary role of lysosomal cathepsins is degradation of extracellular proteins that have been taken up by the cells via pinocytosis or receptor-mediated endocytosis and then transported to the lysosome where they are degraded at the acidic pH values (3-5) in lysosmes. Skeletal muscle has a low level of catheptic enzymes when compared with other tissue such as liver or spleen, and cells, including muscle cells, contain cystatin, a potent inhibitor of cysteine cathepsins in their cytoplasm. Myofibrils are too large to be engulfed by lysosomes (which would result in severing the myofibril and loss of function), so lysosomal cathepsins do not have a primary role in metabolic turnover of myofibrillar proteins, although they may be involved in necrotic degradation, especially during times of macrophage invasion (144-146).
2. The Calpain system. The calpain system includes 14 different members plus calpastatin, a protein inhibitor that is specific for inhibition of the two, ubiquitous, well-characterized calpains, µ-calpain and m-calpain. Skeletal muscle contains appreciable quantities of the calpains, and evidence from a variety of sources indicates that the two ubiquitous calpains have an important role in metabolic turnover of myofibrillar proteins. Members of the NC-1131 Project have examined the Calpain system in the major model animal and livestock species including fish (147; 148).
3. The Proteasome. The proteasome has a major role in intracellular protein turnover in all cells, including muscle cells. The proteasome has a major role in metabolic turnover of the sarcoplasmic protein fraction in skeletal muscle cells, and likely also has a role in turnover of myofibrillar protein (149).
4. The Caspase system. Proteases in the caspase family are responsible for degradation of proteins during apoptosis. The caspases are cysteine proteases, like µ-and m-calpain, but they do not require Ca2+ for activity. Because the caspases are activated only during periods of apoptosis, they do not have a role in the normal metabolic turnover of muscle proteins, although they could conceivably become activated during periods of muscle wasting (150).
Consequently, of the four protease systems in skeletal muscle, only the proteasome and the calpain family are likely to have important roles in metabolic turnover of myofibrillar proteins. The mechanism of how this turnover is accomplished, however, still remains unclear. A plausible mechanism for turnover of the myofibrillar proteins was first proposed nearly 30 years ago (151). It was suggested that the calpains, which are located on the Z-disk and I-band areas of the myofibril (152), cleave those polypeptides, such as titin, nebulin, and desmin that are responsible for maintaining the myofibrillar structure. This cleavage would release the outer layer of filaments from the surface of the myofibril, leaving a myofibril with a diameter that was smaller by one layer or filaments. This model is consistent with observations that atrophying muscle in different muscular dystrophies, after denervation, or during fasting has smaller diameter myofibrils than unaffected muscle has (153).The model is also consistent with the observation that approximately 5-15% of total myofibrillar protein in striated muscle can be released by gentle agitation in an ATP-containing solution (154). The release of these filaments, easily released myofilaments (ERM), does not require hydrolysis of ATP. The amount of ERM increases significantly in the presence of Ca2+, and available evidence indicates that the ERM are an intermediate in the turnover of myofibrillar proteins.
The calpains do not degrade polypeptides to amino acids but do rapidly degrade those proteins that are responsible for maintaining the myofibrillar assembly. The proteasome, on the other hand, cannot degrade intact myofibrils or even intact myofilaments but can rapidly degrade individual polypeptides to small, 4-10-amino acid peptides. Hence, although the mechanism described in the dissociation /proteasome degradation model has been widely quoted as being the mechanism used to turnover myofibrillar proteins in striated muscle, this model has never been critically examined.
Several studies have reported that monomeric actin (155) or myosin molecules (156) can exchange with actin monomers or myosin molecules in myofibrils in the absence of proteolysis, and recently it has been shown that purified alpha-actinin in solution also exchanges with alpha-actinin in myofibrils (157). Therefore, myofibrils may use more than one mechanism to turn over the proteins that constitute the myofibrillar structure. Possibly, those proteins on the surface of the myofibrils, such as the IF protein, turnover via direct exchange, whereas calpain proteolysis is used to release filaments.
Regardless of the mechanism that releases filaments/proteins from the myofibril, it is clear that some other mechanism is needed to degrade the released filaments/proteins to amino acids.
In addition to studying the mechanism of myofibril turnover, the previous NC-1131 project has examined the assembly and organization of the cytoskeleton in developing and adult muscle cells, with emphasis on the proteins associated with the intermediate filament (IF) system. Results obtained during this project demonstrated that IFs have an important role in maintaining the structural integrity of the muscle fiber (158-170). The IFs encircle myofibrils at their Z-lines, link adjacent myofibrils, and help attach the peripheral layer of myofibrils to the cell membrane at sites called costameres. However, the protein interactions involved in organizing the IF network and attaching the IFs to other muscle cell cytoskeletal components are not well characterized. Elucidation of these protein interactions may identify rate-limiting steps in myofibril assembly that influence the rate of muscle growth. Additionally, these studies will contribute to our understanding of molecular interactions affecting meat tenderness.
Researchers in the NC-1131 Project are actively investigating the mechanisms that determine cellular identity/phenotype. Understanding of the mechanisms involved with cellular fate is a critical component of tissue engineering and the potential to control cellular composition of tissues and animals. Investigators are examining the regulation of stem cell fate, along with fat cell and muscle cell interconversion (171-174). Project members are also exploring the role of muscle tissue as a source of stem cells in tissue engineering (175; 176).
As described above, members of the NC-1131 regional project have made significant contributions to our fundamental understanding of the roles of growth factors, cell signaling, protein turnover and myofibril assembly in regulating proliferation, differentiation and growth of muscle cells in meat-producing animals. The studies proposed for the project renewal build upon these findings and will further increase our knowledge of the basic processes regulating muscle growth and differentiation. This fundamental knowledge will provide the framework necessary to develop strategies to increase the efficiency of muscle growth in meat animals.
The following objectives are broadly defined in order to allow for changes in direction of work based on results of current studies both within and outside of the project. Approaches and methodologies are similar to the NC-1131 project but have been changed to reflect advances in knowledge during the five year duration of the currently active project. Because of page limitations general approaches rather than detailed methods are described.
Objectives
-
Characterize the signal transduction pathways that regulate skeletal muscle growth and metabolism including the influence of endogenous growth factors and various production practices..
-
Characterize the cellular and molecular basis of myogenesis.
-
Characterize mechanisms of protein assembly and degradation in skeletal muscle.
Methods
Objective 1: Characterize the signal transduction pathways that regulate skeletal muscle growth and metabolism including the influence of endogenous growth factors and various production practices. Studies in this objective focus primarily on autocrine and paracrine mechanisms regulating muscle cell proliferation and differentiation in economically important animals. Ten stations are involved in studies contributing to this objective. The participating stations are collaborating in two major areas: 1) the role of endogenous growth factors, and 2) the impact of production practices that target muscle growth. Endogenous Growth Factors (Collaborating stations include Arizona, Idaho, Illinois, Indiana, Minnesota, Washington, Wisconsin, and Wyoming): The Arizona Station will examine the role of hepatocyte growth factor (HGF) in activation of quiescent satellite cells in bovine muscle. Initial experiments will examine whether HGF can stimulate activation of quiescent satellite cells in bovine muscle and whether myostatin and TGF-beta1 antagonize this activity. Although evidence suggests that HGF may be the physiological activator of quiescent satellite cells, the hypothesis has not been tested in vivo. Consequently, a second set of experiments will assess whether HGF is responsible for activation of quiescent satellite cells in vivo. Transgenic mice that express a dominant-negative form of HGF in muscle fibers will be generated and satellite cell activation will be examined following muscle injury. It is anticipated that satellite cell activation will be diminished or inhibited following injury in muscle that has expressed the inhibitory dominant negative form of HGF. A third set of experiments will examine what gene expression pattern characterizes the quiescent state of satellite cells and changes in this pattern during activation. Studies at the Illinois station will focus on prenatal interventions which alter muscle growth and metabolism. Hyperplastic capacity of muscle in most vertebrates is determined during embryonic and early post-parturition stages of development, whereas muscle hypertrophy is primarily a postnatal process. Building on previous work using in ovo injection of various hormones and growth factors in chicken eggs, the role of these compounds in establishing muscle fiber type, fiber number, and altering postnatal metabolism will be established. Furthermore, use of mouse models and administration of compounds to the dam during gestation will provide insight into the influence of certain compounds on prenatal development and their ability to alter postnatal growth of offspring. The ability to manipulate fiber number and type will provide tremendous opportunity to improve the efficiency and quality of meat production in our domestic livestock species. The Minnesota Station will continue studies on the biological mechanism by which anabolic steroids enhance muscle growth in cattle and on the mechanism by which insulin-like growth factor binding proteins (IGFBP)-3 and -5 facilitate the proliferation-suppressing actions of myostatin and TGF-beta on myogenic cells from meat-producing animals. Anabolic steroids enhance muscle growth and performance in cattle, however the mechanisms by which they enhance muscle growth are not known. Data obtained in our laboratory show that steroid-enhanced muscle growth in steers involves the interaction of muscle insulin-like growth factor-I (IGF-I) expression, IGF-receptor (IGFR-1), estrogen receptor (ER)-±, G-protein-coupled-receptor-30 (GPR-30), androgen receptor (AR) and specific intracellular signaling pathways. Consequently, our goal is to use combined in vivo and in vitro approaches to define the cellular mechanisms of steroid-enhanced muscle growth in steers. To achieve this goal, we propose to accomplish the following objectives: 1) Assess the interaction of ER-± with IGFR-1 in vitro and in vivo; 2) Assess the individual and combined roles of ER-± and GPR-30 on E2-stimulated IGF-I mRNA expression in vitro and in vivo, and on proliferation, differentiation and(or) protein turnover in vitro; and 3) Assess the involvement of specific intracellular signaling pathways in E2- and TBA-stimulated proliferation and/or IGF-I mRNA expression in BSC. These studies will increase our understanding of the mechanism of anabolic steroid-induced muscle growth in steers and will provide specific information about the interaction of steroids, the IGF system and signaling pathways in this process. We have also shown that both IGFBP-3 and -5 facilitate the proliferation suppressing actions of TGF-beta and myostatin on myogenic cell proliferation; however, the mechanism by which these IGFBPs facilitate TGF-beta and myostatin action is not known. Consequently, we will continue to examine the role of various intracellular signaling molecules and cell surface receptors known to interact with IGFBP-3 and/or -5 to define this mechanism. Because both myostatin and TGF-beta significantly affect muscle growth, increased understanding of the role of IGFBPs in this process will lead to a better understanding of the biological mechanisms controlling muscle growth. Studies at the Wisconsin Station will focus on the role of titin in signal transduction in muscle cells. Wisconsin researchers will examine whether the distributions of titin binding proteins are altered during muscle development. A number of proteins have been shown to bind to titin in cardiac muscle, and many of these same proteins may also interact with the skeletal muscle titin isoforms. Initial studies will be conducted with antibodies against Ankrd2, a protein that binds titin in skeletal muscle and to several transcription factors in the nucleus (177) and muscle LIM protein (177b-177c); in addition these proteins have been shown to re-distribute in response to muscle stretch (177d). Researchers will use Western blotting and immunofluorescence to compare protein expression and localization as they relate to muscle development. Wisconsin researchers hypothesize that the relative content of these proteins will be higher during periods of initial rapid muscle growth and that a larger proportion of these proteins will be found in the nucleus during the rapid early periods of growth. The Washington Station will explore the dedifferentiation of mature adipocytes derived from skeletal muscle adipose depots (intramuscular/marbling fat), and the ability of progeny adipofibroblasts to transdifferentiate into other cell types. Present research has focused on general (comparative) differences between the dedifferentiation of mature, lipid-filled adipocytes from beef cattle and pigs. Interestingly, lipid expulsion is not required for beef-derived adipocytes to resume proliferation, but it is an absolute requirement for pig-derived cells. Moreover, the redifferentiation of cells derived from both animals is quite differentsuggesting that formation of other cell types by transdifferentiation mechanisms is possible. Due to the prevalence of mature cells with the ability to dedifferentiate to form proliferative-competent progeny cells, this system might help explain differences in muscle fatness (hence quality of meat products), diseases like lipidodystrophy whereby muscle mass remains constant but skeletal muscle is replaced by adipose tissue, and other forms of metabolic diseases. Studies at the Idaho Station, in cooperation with the Washington Station, will focus upon leptin effects on partitioning of energy substrate utilization. This effect is to favor oxidation of fatty acids, sparing glucose. Thus, there is a mechanistic link between leptin and insulin in modulating substrate utilization. Researchers will target two mechanisms to identify key signaling molecules in these interactions. 1) Modulation of leptin actions in muscle by leptin binding proteins and other paracrine factors. 2) Activation of common intra-cellular pathways, and key messengers including insulin receptor substrates-1 and -2, phosphatidylinositol-3 kinase, and mitogen-activated phospho-kinase by leptin and insulin. The Idaho Station is will also conduct research to define the population characteristics and cellular and molecular regulation, of post-hatch fish cells. Researchers will 1) isolate representative populations of cells of fish muscle, that are involved in muscle growth, development or quality; 2) define specific cellular and molecular characteristics of the isolated cells and the tissues from which they are derived; and 3) develop viable cell lines for use in studies to define the cellular and molecular regulation of fish growth and development. The Indiana Station will investigate the regulation of satellite cell function by notch signaling in the skeletal muscle. Postnatal muscle growth and hypertrophy requires addition of myonuclei into existing muscle fibers. This process is primarily mediated by muscle specific stem cells called satellite cells. In response to growth signals, quiescent satellite cells are activated, enter the cell cycle and proliferate as myoblasts. Proliferating myoblasts can differentiate into mononuclear muscle cells called myocytes, which then fuse with existing fibers to mediate muscle growth or hypertrophy. Alternatively, proliferating myoblasts can also withdraw from cell cycle, return to quiescence, and self-renew to replenish the satellite cell population. The signaling pathways that regulate the cell fate choice between self-renewal and differentiation of activated satellite cells are not clear. Taking advantage of a transgenic reporter that exhibits green fluorescence (GFP) in cells that receive Notch signaling, the role of Notch signaling transduction in the self-renewal and differentiation of satellite cells will be dissected in vitro and in vivo using single myofiber culture and muscle regeneration models, in which self-renewal and differentiating progenies can be readily identified with specific markers. In addition, satellite cells exhibiting Notch signaling will be purified by Fluorescence Activated Cell Sorting and their gene expression profiles will be analyzed. Furthermore, mouse models that have constitutively activated or de-activated Notch signaling in the satellite cell lineage will be established to examine how perturbations of Notch signaling affect satellite cell function. Together, these studies will reveal critical information regarding the signaling control of satellite cell function and muscle differentiation. The Wyoming station continues to focus on fetal skeletal muscle development. An increasing body of evidence shows that the level of maternal nutrition alters fetal skeletal muscle development with long-term effects on offspring growth and performance. Fetal skeletal muscle development mainly involves myogenesis (muscle cell development), but also involves adipogenesis (adipocyte development) and fibrogenesis (fibroblast development). All these tissues derive from mesenchymal stem cells (MSCs). Shifting the commitment of MSCs from myogenesis to adipogenesis increases intramuscular fat (marbling) improving the quality grade of meats. Results from our laboratory indicate that Wnt/b-catenin signaling regulates MSC differentiation. Wnt/b-catenin up-regulation promotes myogenesis and down-regulation enhances adipogenesis. AMP-activated protein kinase (AMPK) enhances myogenesis at least partially through intensifying b-catenin mediated up-regulation of myogenesis. Inhibition of AMPK promotes adipogenesis in fetal muscle, which should increase marbling. Production Practices that Target Muscle Growth (Collaborating stations include North Carolina, Illinois, Texas and Wyoming): Research at the Illinois Station will examine the mechanisms of action of Beta-adrenergic agonists (BAA) on muscle cell hypertrophy. The live animal growth response and cellular gene expression changes resulting from BAA administration will be determined in rodent and livestock species. Transcriptional and translational level responses will be evaluated through the application of microarray analysis, quantitative PCR, protein level expression and functional analyses. Pathways analysis will allow for the identification of potential targets for improving muscle growth rates and efficiency. Research efforts at the North Carolina Station will be focused on understanding the effects of different nutritional paradigms on skeletal muscle growth in poultry. A traditional poultry management practice has been to delay providing feed to birds until 48 to 72 hours post-hatch. To assess the effects of this practice on muscle growth, research at the North Carolina station is focused on studying the dynamics of satellite cell number/activity and myonuclear apoptosis during different feeding regimens (starvation/dietary lysine levels) over the early post-hatch period in relation to meat yield in poultry (178-181) along with the role of CoQ-10 in regulating muscle energy utilization. The Texas Station will integrate skeletal muscle phenotypic data from a large, 4-generation genomic mapping population of Nellore-Angus crossbred cattle with genome-wide SNP and microarray data to identify genes and gene networks responsible for specific adult skeletal muscle characteristics. At least 60 different phenotypes are collected from these animals and include meat carcass traits, quantitative and sensory panel analyses of steaks for tenderness, flavor, and juiciness, feed efficiency, and others. In particular emphasis will be placed on identifying genetic mechanisms associated with tenderness traits and feed efficiency. In addition, the Texas Station will work with the Wyoming Station to examine the association of alpha-actinin-3 expression in muscle with feed efficiency. Objective 2: Characterize the cellular and molecular basis of myogenesis. Analysis of muscle-specific gene regulation is an active area of research in the agricultural research community and in the larger medical research community. Characterization of the entire sequence of gene regulatory steps that control myogenesis, and characterization of the all of the signaling pathways that regulate muscle-specific genes is clearly beyond the scope of this project. Thus, efforts will be focused on systems that fall within the expertise of the investigators in the NC-1131 team and on systems that are of particular relevance for understanding muscle growth in meat animals. Ten cooperating units will be responsible for the work under this. The goal of the Michigan Station is to identify and characterize specific genes responsible for controlling prenatal myogenesis and postnatal protein accretion in turkey skeletal muscle. Researchers will utilize oligo-nucleotide microarray technology and real-time quantitative PCR (qPCR) approaches to characterize differences in gene expression between two genetic lines of turkeys at various stages of muscle growth and development. Genes which are differentially expressed between the two lines will be selected for further study by the Ohio station using siRNA techniques to characterize the role of each gene in modulating satellite cell proliferation and differentiation. Subsequently, RNA will be isolated from cells from gene knock-down experiments and sent to the Michigan station for microarray and qPCR analysis to further define the effects of regulation of expression of individual genes on total muscle gene expression. Similar experimental approaches will also be applied to characterize gene expression patterns in pig skeletal muscle. Simultaneous identification of differences in gene expression will enable the complex mechanisms controlling muscle development and growth to be deciphered. Work at the Nebraska Station will focus on characterization of muscle proteins associated with calcium regulation in muscle. Researchers will characterize the protein profile of SR-associated proteins in PSE and unaffected (unimproved) meat animals and develop protein microarrays and electrophoretic separation systems for characterization the calcium regulatory proteome. The North Carolina Station is actively focused on studying the dynamics of satellite cell number/activity and myonuclear apoptosis during different feeding regimens over the early embryonic and post-hatch periods. These researchers will examine the proportion of muscle cells lying inside and outside the basal lamina (based upon anti-laminin staining) expressing Pax7, c-met, myoD, and myogenin in relation to mitotic activity (BrdU incorporation). Furthermore, we are actively investigating the role of pre-natal development on post-hatch muscle growth employing transgenic chicken models, and divergent lines of chickens that exhibit vastly different propensities for muscle growth, and ultimate muscle size. Five stations, (Illinois, Indiana, Ohio, South Dakota, and Washington), are studying the mechanism(s) by which the muscle growth inhibitory cytokine, myostatin exerts an effect on muscle growth using three separate approaches. The Washington group is exploiting the fact that some fish species have two myostatin genes. These researchers plan to determine the developmental expression profile of the different myostatin paralogues using molecular techniques and will further test the biological action of these paralogues by generating a myostatin null fish line and by developing transgenic fish overexpressing different dominant-negative myostatin isoforms. Additional studies will employ cloning the myostatin gene from rainbow trout and identifying skeletal muscle-specific transcriptional regulatory elements within the promoters of all three trout paralogues using DNase footprinting and gel-shift assays with nuclear protein from different tissues. Using quite a different approach, the Hawaii Station possesses a transgenic line of mice that over-express the myostatin prodomain. As a result, these mice experience muscle hypertrophy postnatally. These researchers plan to characterize the global gene expression of muscle from these mice and compare it to wild type using microarray analysis which will provide some pictures of what genes or gene groups are regulated by myostatin. Using yet another approach, the South Dakota, Ohio and Arizona Stations have joined efforts to test the hypothesis that satellite cell subpopulations that differ in their responsiveness to growth factors differ in their expression of myostatin and their responsiveness to this polypeptide. These investigators plan to examine the responsiveness of cultured turkey satellite cells and embryonic myoblasts to myostatin during proliferation and differentiation. Muscles differing in contractile and biochemical characteristics will be used to address the effects of muscle type on this process. The Indiana Station has initiated a project to explore the molecular signatures defining satellite cell heterogeneity in fast and slow muscles. They have established unique mouse models that express cyan and red fluorescent proteins in the type I slow and type IIa fast fibers, respectively. These mice provide an unprecedented opportunity to isolate fresh satellite cells from these specific cohorts of fibers and investigate their unique gene expression patterns by high throughput microarray analysis. Furthermore, satellite cells derived from these mice can serve as a great reporter system with which to examine the fiber type differentiation. The MyHC isoforms expressed by newly differentiated myotubes and remodeled myofibers can be readily resolved by their expression of CFP, RFP or neither. Using the classic myostatin null mouse, the Illinois station has identified several muscle transport proteins which are altered subsequent to the loss of myostatin function. Some of these transport proteins have the ability to alter muscle metabolism. It is the aim of the station to further characterize these proteins and the impact on the insulin signaling and fat metabolism of myostatin-null muscle cells. Furthermore, the responsiveness of the hypermuscular myostatin null mouse to other compounds and techniques which increase muscle growth will be determined providing further insight into the role of myostatin in myogenesis and other proteins that perform similar roles during muscle growth. In collaboration with the South Dakota Station, the Ohio Station proposes to continue to explore the involvement of the extracellular matrix in the regulation of muscle development. These scientists plan to determine how syndecan-4 and glypican-1 expression affects the mechanisms of muscle satellite cell proliferation, differentiation, and responsiveness to FGF2 using predominantly turkey or chicken satellite cells. Syndecan-4 and glypican-1 genes will be transfected and overexpressed, or inhibited by siRNA to determine the effect on focal adhesion formation, MAP kinase and protein kinase C alpha pathways. The Wyoming station will examine the role of hedgehog and Wnt signaling pathways in myogenesis in fetal skeletal muscle, and the effect of maternal physiological functions on these pathways and myogenesis from multipotent progenitor cells. Objective 3: Characterize mechanisms of protein assembly and degradation in skeletal muscle. Work will focus on determining the proteolytic mechanisms used to turnover myofibrillar proteins in skeletal muscle of domestic animals and the role of titin and intermediate filaments in the assembly and disassembly of myofibrillar proteins. Four stations will use a coordinated, complementary approach to achieve this objective. The Arizona Station will utilize 3 approaches to determine whether the calpains are involved in generating easily releasable myofilaments (ERM) from myofibrils, both in vitro and in vivo, and then whether the proteasome can degrade the myofilaments released. 1) Cultured myogenic cells incubated with selected inhibitors of the proteasome or the calpains (all studies will use µ- and m-calpain), will be used to determine the effects of inhibitors on the amount of ERM, the level of ubiquitinated myofibrillar proteins, the release of tyrosine (estimate of general protein degradation), and the release of 3-methylhistidine (estimate of myofibrillar protein degradation). 2) Purified myofibrils will be incubated with purified calpain to learn whether calpain can release from these myofibrils myofilaments that can be degraded by the proteasome. 3) Muscle strips obtained from animals that have been treated to increase the rate of myofibrillar protein turnover (unweighting or glucocorticoid administration) will be incubated with proteasome or calpain inhibitors as in Study 1. The Indiana Station will determine whether interaction of intermediate filament (IF) proteins on the myofibrillar with other myofibrillar proteins is altered by posttranslational events such as phosphorylation, whether these events affect the interaction/release of the proteins from the myofibril; whether interaction of alpha-actinin with actin in developing muscle alters interactions of other actin-binding proteins with actin; and whether degradation of specific cytoskeletal proteins changes the rate of exchange of myofibrillar proteins. The Wisconsin Station will examine the assembly of proteins involved in messenger RNA splicing. Proteins of the muscle splicesome will be characterized and potential changes in stoichiometry during growth and development examined using Western blotting, mass spectrometry, and immunofluorescence methods. Primary emphasis will be placed on the SR class of splicing factors.Measurement of Progress and Results
Outputs
- Research supported by this project will result in training of new researchers equipped to use molecular and cell biology methods to study factors regulating proliferation and differentiation of muscle cells in meat-producing animals.
- Elucidation of the autocrine, paracrine and endocrine mechanisms by which specific growth factors such as IGF-I, TGF beta, FGF, HGF, IGFBP and leptin regulate proliferation and differentiation of skeletal muscle cells in meat animals.
- Increased knowledge of the role of specific genes in regulating proliferation and differentiation of muscle cells in meat animals.
- Increased understanding of the role of the extracellular matrix in regulating proliferation and differentiation of muscle cells in meat animals.
- Increased understanding of the role of specific proteases in protein degradation in skeletal muscle.
Outcomes or Projected Impacts
- Publications will be the primary tangible result expected from the project because it focuses on basic research. It is anticipated that utilization of these published results will lead to improved efficiency of lean meat production in domestic livestock and poultry.
- The Committee will sponsor a Symposium on Molecular Mechanisms Regulating Skeletal Muscle Growth and Differentiation. This symposium will provide a mechanism to convey new research information and will increase the visibility of the project.
- Increased understanding of the role of growth factors and cell signaling pathways in regulating proliferation and differentiation of muscle cells may lead to molecular- and cellular biology-based strategies for more efficient production of lean meat which will benefit both producers and consumers. For example, it may be possible to alter fiber type distribution in muscle to increase muscle mass and/or meat quality. Additionally, it may be possible to increase or prolong the proliferative activity of muscle satellite cells so that they provide more DNA to support postnatal muscle fiber growth.
- Elucidation of mechanisms responsible for muscle protein degradation may provide information necessary to utilize molecular- or cell biology-based methods to reduce protein degradation in growing muscle. This will allow more rapid muscle growth without increasing energy requirements.
- Gene expression studies may lead to identification of genes that may be used to produce transgenic animals that more efficiently produce muscle. Additionally, because this project combines the skills of muscle biologists and molecular biologists, collaboration between committee members should result in elucidation of the function in muscle growth and differentiation of proteins produced by identified genes.
Milestones
(2014): Sponsor a symposium at ASAS/ADSA National Meeting on Cellular Mechanisms Regulating Skeletal Muscle Growth and the Impact on Sustainable Agriculture. This will allow us to convey research findings to interested individuals in these organizations.Projected Participation
View Appendix E: ParticipationOutreach Plan
Dissemination of the results of the research will be done via the timely publication of refereed articles in established journals. Because this a basic research project, publications will be the primary tangible result expected from the project. It is anticipated that utilization of these published results will lead to improved efficiency of lean meat production in domestic livestock and poultry. Additionally, the committee will organize a symposium on Cellular Mechanisms Regulating Skeletal Muscle Growth and the Impact on Sustainable Agriculture at the ASAS/ADSA National Meeting that will convey recent research findings to the members of these organizations.
Organization/Governance
The Technical Committee will consist of at least one representative from each participating unit, appointed as described in the Manual for Cooperative Regional Research, with one representative from each unit designated as the voting member. The North Central Regional Association of Directors will appoint one Director to serve as Administrative Advisor and a representative of the USDA/CSREES will also serve as an Advisor; both will be ex officio member of this committee. The Technical Committee will elect a Chair and a Secretary to serve for a period of one year, and these officers will continue to be voting members. In succeeding years, the Secretary will become Chair, and only a new Secretary will be elected. The Chair, the Secretary, the immediate Past Chair and the Administrative Advisor will serve as the Executive Committee, and they will have the authority to act on behalf of the Technical Committee during the periods between meetings. Because this is a proposal for renewal of the NC-1131 project, the current Chair will serve as Past Chair for the first year of the revised project and the current Secretary will become Chair if the renewal is approved.
The Chair, with approval of the Administrative Advisor, will call yearly meetings of the Technical Committee and interim meetings of the Executive Committee if needed. A report of research results from each unit will be presented orally and in writing at the Technical Committee meetings. The written report should include a list of publications resulting from research related to the project for the year. Reports will be critically reviewed by the Technical Committee and recommendations will be made for future research and coordination of research between units to maximize attainment of the Objectives. The Secretary will prepare minutes and an Annual Report for distribution to Technical Committee members, Directors and Department Chairs of participating State Agricultural Experiment Stations and USDA Laboratories, and the Regional Research Office, CSREES. Because submission of a yearly progress report is essential to assess progress, ensure participation, and prepare the Annual Report, any unit that does not submit a written progress report for two consecutive years will be considered not to be participating and will be dropped from the project.
A station, agency, or institution not currently listed as a participant in the project can petition to join by submitting an addendum to the Methods section of this proposal. This addendum should describe the proposed work and how it contributes to the project. The Administrative Advisor will circulate the addendum to the voting members of the Technical Committee for their consideration and approval. If approved by a majority of the voting members of the Technical Committee, the addendum will be added to the official project outline.
Literature Cited
1. Kamanga-Sollo, E., M. S. Pampusch, G. Xi, M. E. White, M. R. Hathaway, and W. R. Dayton. 2004. IGF-I mRNA levels in bovine satellite cell cultures: Effects of fusion and anabolic steroid treatment. J. Cell Physiol 201:181-189.
2. Pampusch, M. S., B. J. Johnson, M. E. White, M. R. Hathaway, J. D. Dunn, A. T. Waylan, and W. R. Dayton. 2003. Time course of changes in growth factor mRNA levels in muscle of steroid-implanted and nonimplanted steers. J. Anim Sci. 81:2733-2740.
3. Schoonmaker, J. P., S. C. Loerch, F. L. Fluharty, T. B. Turner, S. J. Moeller, J. E. Rossi, W. R. Dayton, M. R. Hathaway, and D. M. Wulf. 2002. Effect of an accelerated finishing program on performance, carcass characteristics, and circulating insulin-like growth factor I concentration of early-weaned bulls and steers. J. Anim Sci. 80:900-910.
4. White, M. E., B. J. Johnson, M. R. Hathaway, and W. R. Dayton. 2003. Growth factor messenger RNA levels in muscle and liver of steroid-implanted and nonimplanted steers. J. Anim Sci. 81:965-972.
5. Hathaway, M. R., W. R. Dayton, M. E. White, and M. S. Pampusch. 2003. Effects of antimicrobials and weaning on porcine serum insulin-like growth factor binding protein levels. J. Anim Sci. 81:1456-1463.
6. Schoonmaker, J. P., S. C. Loerch, F. L. Fluharty, T. B. Turner, S. J. Moeller, J. E. Rossi, W. R. Dayton, M. R. Hathaway, and D. M. Wulf. 2002. Effect of an accelerated finishing program on performance, carcass characteristics, and circulating insulin-like growth factor I concentration of early-weaned bulls and steers. J Anim Sci. 80:900-910.
7. Johnson, B. J., N. Halstead, M. E. White, M. R. Hathaway, and W. R. Dayton. 1998. Activation State of Muscle Satellite Cells Isolated from Steers Implanted with a Combined Trenbolone Acetate and Estradiol Implant. J. Anim. Sci. 76:2779-2786.
8. Yang, F., B. J. Johnson, M. E. White, M. R. Hathaway, and W. R. Dayton. 1999. Effect of insulin-like growth factor (IGF)-I and Des (1-3) IGF-I on the level of IGF binding protein-3 and IGF binding protein -3 mRNA in cultured porcine embryonic muscle cells. J. Cell. Physiol. 178:227-234.
9. Schneider, F., E. Kanitz, D. E. Gerrard, G. Kuhn, K. P. Brussow, K. Nurnberg, I. Fiedler, G. Nurnberg, K. Ender, and C. Rehfeldt. 2002. Administration of recombinant porcine somatotropin (rpST) changes hormone and metabolic status during early pregnancy. Domest. Anim Endocrinol. 23:455-474.
10. Gahr, S.A., Kocamis, J.J., J. Killefer. The effects of in ovo rhIGF-1 administration oin expression of the GH secretagogue receptor (GHSR) during chicken embryonic development. Growth, Development and Aging. 68:3-10, 2004.
11. Xi, G., E. Kamanga-Sollo, M. R. Hathaway, W. R. Dayton, and M. E. White. 2006. Effect of constitutive expression of porcine IGFBP-3 on proliferation and differentiation of L6 myogenic cells. Domest. Anim Endocrinol. 31:35-51.
12. Han, B. , Junfeng Tong, C. Ma, M. J. Zhu, and M. Du. 2008. Insulin-like growth factor-1 (IGF-1) and leucine stimulate mammalian target of rapamycin (mTOR) signaling in pig myogenic satellite cells. Molecular Reproduction and Development, 75: 810-817.
13. McFarland, D. C., X. Liu, S. G. Velleman, C. Zeng, C. S. Coy, and J. E. Pesall. 2003. Variation in fibroblast growth factor response and heparan sulfate proteoglycan production in satellite cell populations. Comp Biochem. Physiol C. Toxicol. Pharmacol. 134:341-351.
14. Liu, X., D. C. McFarland, K. E. Nestor, and S. G. Velleman. 2003. Expression of fibroblast growth factor 2 and its receptor during skeletal muscle development from turkeys with different growth rates. Domest. Anim Endocrinol. 25:215-229.
15. Jin, H. J., M. A. Dunn, D. Borthakur, and Y. S. Kim. 2004. Refolding and purification of unprocessed porcine myostatin expressed in Escherichia coli. Protein Expr. Purif. 35:1-10.
16. Kamanga-Sollo, E., M. S. Pampusch, M. E. White, and W. R. Dayton. 2003. Role of insulin-like growth factor binding protein (IGFBP)-3 in TGF-beta- and GDF-8 (myostatin)-induced suppression of proliferation in porcine embryonic myogenic cell cultures. J. Cell Physiol 197:225-231.
17. Kim, Y.S., N.K. Bobilli, K.S. Paek and H.J. Jin. 2006. In-ovo administration of monoclonal anti-myostatin antibody improves post-hatch chicken growth and muscle mass. Poultry Science 85:1062-1071.
18. McFarland, Douglas C., Sandra G. Velleman, Jane E. Pesall, and Caini Liu. 2006. Effect of Myostatin on Turkey Myogenic Satellite Cells and Embryonic Myoblasts. Comparative Biochemistry and Physiology 144(4): 501-508.
19. Kim, Y.S., N.K. Bobbili, Y.K. Lee and M. Dunn. 2007. Production of a polyclonal antibody against unprocessed chicken myostatin and the effects of in-ovo administration of the antibody on post-hatch broiler growth and muscle mass. Poult. Sci. 86:1196-1205
20. McFarland, D.C., Velleman, S.G., Pesall, J.E., and Liu, C. 2006. Effect of myostatin on turkey myogenic satellite cells and embryonic myoblasts. Comp. Biochem. Physiol. Pt A 144:501-508.
21. Allen, D. L., and M. Du. 2008. Comparative functional analysis of the cow and mouse myostatin genes reveals novel regulatory elements in their upstream promoter regions. Comparative Biochemistry and Physiology, 150: 432-439.
22. Lee, S.B., Y.S. Kim and H.J. Jin. 2007. Characterization and expression pattern of myostatin in the rockfish, Sebastes schlegeli. J. Fisheries Sci. Technol. 10:60-67.
23. Johnson, B. J., M. E. White, M. R. Hathaway, C. J. Christians, and W. R. Dayton. 1998. Effect of a combined trenbolone acetate and estradiol implant on steady-state IGF-I mRNA Concentrations in the liver of wethers and the longissimus muscle of steers. J. Anim. Sci. 76:491-497.
24. Tatsumi, R., A. Hattori, Y. Ikeuchi, J. E. Anderson, and R. E. Allen. 2002. Release of hepatocyte growth factor from mechanically stretched skeletal muscle satellite cells and role of pH and nitric oxide. Mol. Biol. Cell 13:2909-2918.
25. Tatsumi, R., S. M. Sheehan, H. Iwasaki, A. Hattori, and R. E. Allen. 2001. Mechanical stretch induces activation of skeletal muscle satellite cells in vitro. Exp. Cell Res. 267:107-114.
26. Sheehan, S. M., R. Tatsumi, C. J. Temm-Grove, and R. E. Allen. 2000. HGF is an autocrine growth factor for skeletal muscle satellite cells in vitro. Muscle Nerve 23:239-245.
27. Sheehan, S. M. and R. E. Allen. 1999. Skeletal muscle satellite cell proliferation in response to members of the fibroblast growth factor family and hepatocyte growth factor. J. Cell. Physiol. 181:499-506.
28. Tatsumi, R. and R. E. Allen. 2004. Active hepatocyte growth factor is present in skeletal muscle extracellular matrix. Muscle Nerve 30:654-658.
29. Hill, R. A., A. L. Strat, N. J. Hughes, T. J. Kokta, M. V. Dodson, and A. Gertler. 2004. Early insulin signaling cascade in a model of oxidative skeletal muscle: mouse Sol8 cell line. Biochim. Biophys. Acta 1693:205-211.
30. Margetic, S., C. Gazzola, G. G. Pegg, and R. A. Hill. 2002. Characterization of leptin binding in bovine kidney membranes. Domest. Anim Endocrinol. 23:411-424.
31. Margetic, S., C. Gazzola, G. G. Pegg, and R. A. Hill. 2002. Leptin: a review of its peripheral actions and interactions. Int. J. Obes. Relat Metab Disord. 26:1407-1433.
32. Strat, A.L., Kokta, T.A., Dodson, M.V., Gertler, A., Wu, Z. and Hill, R.A. (2005). Early Signaling Interactions between the Insulin and Leptin Pathways in Bovine Myogenic Cells. Biochimica et Biophysica Acta 1744:164-175.
33. Forhead, A.J., C. A. Lamb, K. L. Franko, D. M. O'Connor, F. B. P. Wooding, R. L. Cripps, S. Ozanne, D. Blache, Q. W Shen, M. Du, and A. L. Fowden. 2008. Role of leptin in the regulation of growth and carbohydrate metabolism in the ovine fetus during late gestation. Journal of Physiology, 586: 2393-2403.
34. Xi, G., E. Kamanga-Sollo, M. S. Pampusch, M. E. White, M. R. Hathaway, and W. R. Dayton. Effect of recombinant porcine IGFBP-3 on IGF-I and Long-R3-IGF-I-stimulated proliferation and differentiation of L6 myogenic cells. Journal of Cellular Physiology 200, 387-394. 2004.
35. Hathaway, M. R., W. R. Dayton, M. E. White, T. L. Henderson, D. A. Young, and T. N. Doan. 1999. Effect of feed intake on antimicrobially induced increases in porcine serum insulin-like growth factor I. J. Anim Sci. 77:3208-3214.
36. Yi, Z., M. R. Hathaway, W. R. Dayton, and M. E. White. 2001. Effects of growth factors on insulin-like growth factor binding protein (IGFBP) secretion by primary porcine satellite cell cultures. J. Anim Sci. 79:2820-2826.
37. Pampusch, M. S., E. Kamanga-Sollo, M. E. White, M. R. Hathaway, and W. R. Dayton. 2003. Effect of recombinant porcine IGF-binding protein-3 on proliferation of embryonic porcine myogenic cell cultures in the presence and absence of IGF-I. J. Endocrinol. 176:227-235.
38. Johnson, B. J., M. E. White, M. R. Hathaway, and W. R. Dayton. 2003. Effect of differentiation on levels of insulin-like growth factor binding protein mRNAs in cultured porcine embryonic myogenic cells. Domest. Anim Endocrinol. 24:81-93.
39. Johnson, B. J., M. E. White, M. R. Hathaway, and W. R. Dayton. 1999. Decreased steady-state insulin-like growth factor binding protein-3 (IGFBP-3) mRNA level is associated with differentiation of cultured porcine myogenic cells. J. Cell. Physiol. 179:237-243.
40. Li, J. and Johnson, S. E. 2006. ERK2 is required for efficient terminal differentiation of skeletal myoblasts. Biochem. Biophys. Res. Commun. 345(4):1425-1433.
41. Reed SA, Ouellette SE, Liu X, Allen RE, Johnson SE. (2007). E2F5 and LEK1 translocation to the nucleus is an early event demarcating myoblast quiescence. J Cell Biochem.101:1394 408.
42. Du, M., Q. W. Shen, M. J. Zhu, and S. P. Ford. 2007. Leucine stimulates mTOR signalling in C2C12 myoblast cells through inhibition of AMP-activated protein kinase. J. Anim. Sci. 85: 919-927.
43. Shen, Q. W., K. R. Underwood, W. J. Means, R. J. McCormick, and M. Du. 2007. Halothane gene, energy metabolism, AMP-activated protein kinase, and glycolysis in postmortem pig longissimus dorsi muscle. J. Anim. Sci. 85: 1054-1061.
44. Shen, Q.W., D.E. Gerrard and M. Du. 2008. Compound C, an inhibitor of AMP-activated protein kinase, inhibits glycolysis in mouse Longissimus Dorsi postmortem. Meat Sci. 78(3) p. 323-330.
45. Tong, J., M. J. Zhu, K. R. Underwood, B. W. Hess, S. P. Ford, and M. Du. 2008. AMP-activated protein kinase negatively regulates adipogenesis in sheep fetal skeletal muscle and 3T3 cells. Journal of Animal Science, 86: 1296-1305.
46. Shi, H., C. Zeng A. Ricome, K.M. Hannon, A.L. Grant and D.E. Gerrard. 2007. Extracellular signal regulated kinase pathway is differentially involved in beta-agonist-induced hypertrophy in slow and fast muscles. Am. J. Physiol. Cell Physiol. 292: C1681-C1689.
47. Sissom, E. K., C. D. Reinhardt, J. P. Hutcheson, W. T. Nichols, D. A. Yates, R. S. Swingle, and B. J. Johnson. 2007. Response to ractopamine-HCl in heifers is altered by implant strategy across days on feed. J. Anim. Sci. 85:2125-2132.
48. Kambadur, R., M. Sharma, T. P. Smith, and J. J. Bass. 1997. Mutations in myostatin (GDF8) in double-muscled Belgian Blue and Piedmontese cattle. Genome. Res. 7:910-916.
49. Grobet, L., L. J. Martin, D. Poncelet, D. Pirottin, B. Brouwers, J. Riquet, A. Schoeberlein, S. Dunner, F. Menissier, J. Massabanda, R. Fries, R. Hanset, and M. Georges. 1997. A deletion in the bovine myostatin gene causes the double-muscled phenotype in cattle. Nat. Genet. 17:71-74.
50. Johnson, B. J., P. T. Anderson, J. C. Meiske, and W. R. Dayton. 1996. Effect of a combined trenbolone acetate and estradiol implant on steroid hormone levels, feedlot performance, carcass characteristics and carcass composition of feedlot steers. J. Anim. Sci. 74:363-371.
51. Underwood, L. E. 1996. Nutritional regulation of IGF-I and IGFBPs. J. Pediatr. Endocrinol. Metab. 9 Suppl 3:303-12:303-312.
52. Barton-Davis, E. R., D. I. Shoturma, A. Musaro, N. Rosenthal, and H. L. Sweeney. 1998. Viral mediated expression of insulin-like growth factor I blocks the aging-related loss of skeletal muscle function. Proc. Natl. Acad. Sci. USA 95:15603-15607.
53. Grant, A. L., W. G. Helferich, S. A. Kramer, R. A. Merkel, and W. G. Bergen. 1991. Administration of growth hormone to pigs alters the relative amount of insulin-like growth factor-I mRNA in liver and skeletal muscle. J. Endocrinol. 130:331-338.
54. Galvin, C. D., O. Hardiman, and C. M. Nolan. 2003. IGF-1 receptor mediates differentiation of primary cultures of mouse skeletal myoblasts. Mol. Cell Endocrinol 200:19-29.
55. Broussard, S. R., R. H. McCusker, J. E. Novakofski, K. Strle, W. H. Shen, R. W. Johnson, G. G. Freund, R. Dantzer, and K. W. Kelley. 2003. Cytokine-hormone interactions: tumor necrosis factor alpha impairs biologic activity and downstream activation signals of the insulin-like growth factor I receptor in myoblasts. Endocrinology 144:2988-2996.
56. Wilson, E. M., M. M. Hsieh, and P. Rotwein. 2003. Autocrine growth factor signaling by insulin-like growth factor-II mediates MyoD-stimulated myocyte maturation. J. Biol. Chem. 278:41109-41113.
57. McCusker, R. H. and J. Novakofski. 2003. Zinc partitions insulin-like growth factors (IGFs) from soluble IGF binding protein (IGFBP)-5 to the cell surface receptors of BC3H-1 muscle cells. J. Cell Physiol 197:388-399.
58. Salih, D. A., G. Tripathi, C. Holding, T. A. Szestak, M. I. Gonzalez, E. J. Carter, L. J. Cobb, J. E. Eisemann, and J. M. Pell. 2004. Insulin-like growth factor-binding protein 5 (Igfbp5) compromises survival, growth, muscle development, and fertility in mice. Proc. Natl. Acad. Sci. U. S. A 101:4314-4319.
59. Foulstone, E. J., P. B. Savage, A. L. Crown, J. M. Holly, and C. E. Stewart. 2003. Role of insulin-like growth factor binding protein-3 (IGFBP-3) in the differentiation of primary human adult skeletal myoblasts. J. Cell Physiol 195:70-79.
60. Rotwein, P., P. L. James, and K. Kou. 1995. Rapid activation of insulin-like growth factor binding protein-5 gene transcription during myoblast differentiation. Mol. Endocrinol. 9:913-923.
61. Han, J.-K. and G. R. Martin. 1993. Embryonic expression of Fgf-6 is restricted to the skeletal muscle lineage. Develop. Biol. 158:549-554.
62. McPherron, A. C., A. M. Lawler, and S. J. Lee. 1997. Regulation of skeletal muscle mass in mice by a new TGF-b superfamily member. Nature 387:83-90.
63. Bosche, W. J., D. Z. Ewton, and J. R. Florini. 1995. Transforming growth factor-beta isoform expression in insulin- like growth factor stimulated myogenesis. J. Cell. Physiol. 164:324-333.
64. Foulstone, E. J., C. Huser, A. L. Crown, J. M. Holly, and C. E. Stewart. 2004. Differential signalling mechanisms predisposing primary human skeletal muscle cells to altered proliferation and differentiation: roles of IGF-I and TNFalpha. Exp. Cell Res. 294:223-235.
65. Halevy, O. and L. C. Cantley. 2004. Differential regulation of the phosphoinositide 3-kinase and MAP kinase pathways by hepatocyte growth factor vs. insulin-like growth factor-I in myogenic cells. Exp. Cell Res. 297:224-234.
66. Lawlor, M. A., X. Feng, D. R. Everding, K. Sieger, C. E. Stewart, and P. Rotwein. 2000. Dual control of muscle cell survival by distinct growth factor-regulated signaling pathways. Mol. Cell Biol. 20:3256-3265.
67. Li, Y., B. Jiang, W. Y. Ensign, P. K. Vogt, and J. Han. 2000. Myogenic differentiation requires signalling through both phosphatidylinositol 3-kinase and p38 MAP kinase. Cell Signal. 12:751-757.
68. Gonzalez, I., G. Tripathi, E. J. Carter, L. J. Cobb, D. A. Salih, F. A. Lovett, C. Holding, and J. M. Pell. 2004. Akt2, a novel functional link between p38 mitogen-activated protein kinase and phosphatidylinositol 3-kinase pathways in myogenesis. Mol. Cell Biol. 24:3607-3622.
69. Kaliman, P., J. Canicio, P. R. Shepherd, C. A. Beeton, X. Testar, M. Palacin, and A. Zorzano. 1998. Insulin-like growth factors require phosphatidylinositol 3-kinase to signal myogenesis: dominant negative p85 expression blocks differentiation of L6E9 muscle cells. Mol. Endocrinol. 12:66-77.
70. Al Khalili, L., A. V. Chibalin, M. Yu, B. Sjodin, C. Nylen, J. R. Zierath, and A. Krook. 2004. MEF2 activation in differentiated primary human skeletal muscle cultures requires coordinated involvement of parallel pathways. Am. J. Physiol Cell Physiol 286:C1410-C1416.
71. Coolican, S. A., D. S. Samuel, D. Z. Ewton, F. J. McWade, and J. R. Florini. 1997. The mitogenic and myogenic actions of insulin-like growth factors utilize distinct signaling pathways. J. Biol. Chem. 272:6653-6662.
72. Deng, X., D. Z. Ewton, B. Pawlikowski, M. Maimone, and E. Friedman. 2003. Mirk/dyrk1B is a Rho-induced kinase active in skeletal muscle differentiation. J. Biol. Chem. 278:41347-41354.
73. Zhu, S., P. J. Goldschmidt-Clermont, and C. Dong. 2004. Transforming growth factor-beta-induced inhibition of myogenesis is mediated through Smad pathway and is modulated by microtubule dynamic stability. Circ. Res. 94:617-625.
74. Liu, D., J. S. Kang, and R. Derynck. 2004. TGF-beta-activated Smad3 represses MEF2-dependent transcription in myogenic differentiation. EMBO J. 23:1557-1566.
75. Joulia, D., H. Bernardi, V. Garandel, F. Rabenoelina, B. Vernus, and G. Cabello. 2003. Mechanisms involved in the inhibition of myoblast proliferation and differentiation by myostatin. Exp. Cell Res. 286:263-275.
76. Miller, M. K., H. Granzier, E. Ehler, and C. C. Gregorio. 2004. The sensitive giant: the role of titin-based stretch sensing complexes in the heart. Trends Cell Biol. 14:119-126.
77. Tatsumi, R., X. Liu, A. Pulido, M. Morales, T. Sakata, S. Dial, A. Hattori, Y. Ikeuchi, and R.E. Allen. 2006. Satellite cell activation in stretched skeletal muscle and the role of nitric oxide and hepatocyte growth factor. Am. J. Physiol. Cell Physiol. 290:C1487-94.
78. Yamada, M., R. Tatsumi, T. Kikuri, S. Okamoto, S. Nonoshita, W. Mizunoya, Y. Ikeuchi, H. Shimokawa, K. Sunagawa, and R.E. Allen. 2006. Matrix metalloproteinases are involved in mechanical stretch-induced activation of skeletal muscle satellite cells. Muscle Nerve 34:313-319.
79. Yamada, M., Y. Sankoda, R. Tatsumi, W. Mizunoya, Y. Ikeuchi, K. Sunagawa, and R. E. Allen. 2008. Matrix metalloproteinase-2 mediates stretch-induced activation of skeletal muscle satellite cells in a nitric oxide-dependent manner. Int. J. Biochem. Cell Biol. 40(10):2183-91.
80. Kramerova, I., E. Kudryashova, J. G. Tidball, and M. J. Spencer. 2004. Null mutation of calpain 3 (p94) in mice causes abnormal sarcomere formation in vivo and in vitro. Hum. Mol. Genet. 13:1373-1388.
81. Wang, X., S. R. Thomson, J. D. Starkey, J. L. Page, A. D. Ealy, and S. E. Johnson. 2004. Transforming growth factor beta1 is up-regulated by activated Raf in skeletal myoblasts but does not contribute to the differentiation-defective phenotype. J. Biol. Chem. 279:2528-2534.
82. Page, J. L., X. Wang, L. M. Sordillo, and S. E. Johnson. 2004. MEKK1 signaling through p38 leads to transcriptional inactivation of E47 and repression of skeletal myogenesis. J. Biol. Chem. 279:30966-30972.
83. Johnson, S. E., C. M. Dorman, and S. A. Bolanowski. 2002. Inhibition of myogenin expression by activated Raf is not responsible for the block to avian myogenesis. J. Biol. Chem. 277:28742-28748.
84. Kamanga-Sollo, E., M. S. Pampusch, M. E. White, and W. R. Dayton. 2003. Role of insulin-like growth factor binding protein (IGFBP)-3 in TGF-beta- and GDF-8 (myostatin)-induced suppression of proliferation in porcine embryonic myogenic cell cultures. J. Cell Physiol 197:225-231.
85. Liu, X., K. E. Nestor, D. C. McFarland, and S. G. Velleman. 2002. Developmental expression of skeletal muscle heparan sulfate proteoglycans in turkeys with different growth rates. Poult. Sci. 81:1621-1628.
86. Liu, X., D. C. McFarland, K. E. Nestor, and S. G. Velleman. 2004. Developmental regulated expression of syndecan-1 and glypican in pectoralis major muscle in turkeys with different growth rates. Dev. Growth Differ. 46:37-51.
87. Velleman, S. G. 2000. The role of the extracellular matrix in skeletal development. Poult. Sci. 79:985-989.
88. Velleman, S. G., C. S. Coy, L. Gannon, M. Wick, and D. C. McFarland. 2000. Beta1 integrin expression during normal and low score normal avian myogenesis. Poult. Sci. 79:1179-1182.
89. Velleman, S. G. and D. C. McFarland. 2004. Beta1 integrin mediation of myogenic differentiation: implications for satellite cell differentiation. Poult. Sci. 83:245-252.
90. Velleman, S. G., X. Liu, C. S. Coy, and D. C. McFarland. 2004. Effects of syndecan-1 and glypican on muscle cell proliferation and differentiation: implications for possible functions during myogenesis. Poult. Sci. 83:1020-1027.
91. Zimmerman, S. D., D. P. Thomas, S. G. Velleman, X. Li, T. R. Hansen, and R. J. McCormick. 2001. Time course of collagen and decorin changes in rat cardiac and skeletal muscle post-MI. Am. J. Physiol Heart Circ. Physiol 281:H1816-H1822.
92. Velleman, S.G., and Mozdziak, P.E. 2005. Effects of posthatch feed deprivation on heparan sulfate proteoglycan, syndecan-1, and glypican expression: Implications for muscle growth potential in chickens. Poult. Sci. 84:601-606.
93. Velleman, S.G., and Nestor, K.E. 2005. Effect of genetic increases in egg production, age, and sex on muscle development in turkeys. Poult Sci. 84:1347-1349.
94. Li, X., McFarland, D.C., and Velleman, S.G. 2006. Effect of transforming growth factor beta on decorin and beta 1 integrin expression during muscle development in chickens. Poult. Sci 85:326-332.
95. Velleman, S.G., Liu, C., Coy, C.S., and McFarland, D.C. 2006. Effects of glypican-1 on turkey skeletal muscle cell proliferation, differentiation, and fibroblast growth factor 2 responsiveness. Dev. Growth Diff. 48:271-276.
96. Velleman, S.G., Coy, C.S., and McFarland, D.C. 2007. Effect of syndecan-1, syndecan-4, and glypican-1 on turkey muscle satellite cell proliferation, differentiation, and responsiveness to fibroblast growth factor 2. Poult. Sci. 86:1406-1413.
97. Li, X., D.C. McFarland, and S.G. Velleman. 2008. Extracellular matrix proteoglycan decorin-mediated myogenic satellite cell responsiveness to transforming growth factor-beta during satellite cell proliferation and differentiation. Dom. Anim. Endocrinol. 35:263-273.
98. Melo, F., D. J. Carey, and E. Brandan. 1996. Extracellular matrix is required for skeletal muscle differentiation but not myogenin expression. J. Cell Biochem. 62:227-239.
99. Osses, N. and E. Brandan. 2002. ECM is required for skeletal muscle differentiation independently of muscle regulatory factor expression. Am. J. Physiol Cell Physiol 282:C383-C394.
100. Isaka, Y., D. K. Brees, K. Ikegaya, Y. Kaneda, E. Imai, N. A. Noble, and W. A. Border. 1996. Gene therapy by skeletal muscle expression of decorin prevents fibrotic disease in rat kidney. Nat. Med. 2:418-423.
101. Iozzo, R. V. 1997. The family of the small leucine-rich proteoglycans: key regulators of matrix assembly and cellular growth. Crit Rev. Biochem. Mol. Biol. 32:141-174.
102. Riquelme, C., J. Larrain, E. Schonherr, J. P. Henriquez, H. Kresse, and E. Brandan. 2001. Antisense inhibition of decorin expression in myoblasts decreases cell responsiveness to transforming growth factor beta and accelerates skeletal muscle differentiation. J. Biol. Chem. 276:3589-3596.
103. Fuentealba, L., D. J. Carey, and E. Brandan. 1999. Antisense inhibition of syndecan-3 expression during skeletal muscle differentiation accelerates myogenesis through a basic fibroblast growth factor-dependent mechanism. J. Biol. Chem. 274:37876-37884.
104. Larrain, J., D. J. Carey, and E. Brandan. 1998. Syndecan-1 expression inhibits myoblast differentiation through a basic fibroblast growth factor-dependent mechanism. J. Biol. Chem. 273:32288-32296.
105. Olwin, B. B. and A. Rapraeger. 1992. Repression of myogenic differentiation by aFGF, bFGF, and K-FGF is dependent on cellular heparan sulfate. J. Cell Biol. 118:631-639.
106. Naka, D., T. Ishii, T. Shimomura, T. Hishida, and H. Hara. 1993. Heparin modulates the receptor-binding and mitogenic activity of hepatocyte growth factor on hepatocytes. Exp. Cell Res. 209:317-324.
107. Lopez-Casillas, F., C. Riquelme, Y. Perez-Kato, M. V. Ponce-Castaneda, N. Osses, J. Esparza-Lopez, G. Gonzalez-Nunez, C. Cabello-Verrugio, V. Mendoza, V. Troncoso, and E. Brandan. 2003. Betaglycan expression is transcriptionally up-regulated during skeletal muscle differentiation. Cloning of murine betaglycan gene promoter and its modulation by MyoD, retinoic acid, and transforming growth factor-beta. J. Biol. Chem. 278:382-390.
108. Olson, E. N. 1990. MyoD family: a paradigm for development? Genes Dev. 4:1454-1461.
109. Weintraub, H., R. Davis, S. Tapscott, M. Thayer, M. Krause, R. Benezra, T. K. Blackwell, D. Turner, R. Rupp, S. Hollenberg, Y. Zhuang, and A. Lassar. 1991. The myoD gene family: Nodal point during specification of the muscle cell lineage. Science 251:761-766.
110. Weintraub, H. 1993. The MyoD family and myogenesis: Redundancy, networks, and thresholds. Cell 75:1241-1244.
111. Davis, R. L., H. Weintraub, and A. B. Lassar. 1987. Expression of a single transfected cDNA converts fibroblasts to myoblasts. Cell 51:987-1000.
112. Edmondson, D. G. and E. N. Olson. 1989. A gene with homology to the myc similarity region of MyoD1 is expressed during myogenesis and is sufficient to activate the muscle differentiation program. Genes Dev. 3:628-640.
113. Wright, W. E., D. A. Sassoon, and V. K. Lin. 1989. Myogenin, a factor regulating myogenesis, has a domain homologous to MyoD. Cell 56:607-617.
114. Braun, T., G. Buschhausen-Denker, E. Bober, E. Tannich, and H. H. Arnold. 1989. A novel human muscle factor related to but distinct from MyoD1 induces myogenic conversion in 10T1/2 fibroblasts. EMBO J. 8:701-709.
115. Braun, T., E. Bober, B. Winter, N. Rosenthal, and H. H. Arnold. 1990. Myf-6, a new member of the human gene family of myogenic determination factors: Evidence for a gene cluster on chromosome 12. EMBO J. 9:821-831.
116. Miner, J. H. and B. Wold. 1990. Herculin, a fourth member of the MyoD family of myogenic regulatory genes. Proc. Natl. Acad. Sci. U. S. A 87:1089-1093.
117. Rhodes, S. J. and S. F. Konieczny. 1989. Identification of MRF4: A new member of the muscle regulatory factor gene family. Genes Dev. 3:2050-2061.
118. Brennan, T. J. and E. N. Olson. 1990. Myogenin resides in the nucleus and acquires high affinity for a conserved enhancer element on heterodimerization. Genes Dev. 4:582-595.
119. Lassar, A. B., R. L. Davis, W. E. Wright, T. Kadesch, C. Murre, A. Voronova, D. Baltimore, and H. Weintraub. 1991. Functional activity of myogenic HLH proteins requires hetero-oligomerization with E12/E47-like proteins in vivo. Cell 66:305-315.
120. Roy, K., l. S. de, I, and A. N. Imbalzano. 2002. The myogenic basic helix-loop-helix family of transcription factors shows similar requirements for SWI/SNF chromatin remodeling enzymes during muscle differentiation in culture. J. Biol. Chem. 277:33818-33824.
121. Braun, T., B. Winter, E. Bober, and H. H. Arnold. 1990. Transcriptional activation domain of the muscle-specific gene-regulatory protein myf5. Nature 346:663-665.
122. Lassar, A. B., J. N. Buskin, D. Lockshon, R. L. Davis, S. Apone, S. D. Hauschka, and H. Weintraub. 1989. MyoD is a sequence-specific DNA binding protein requiring a region of myc homology to bind to the muscle creatine kinase enhancer. Cell 58:823-831.
123. Lin, H., K. E. Yutzey, and S. F. Konieczny. 1991. Muscle-specific expression of the troponin I gene requires interactions between helix-loop-helix muscle regulatory factors and ubiquitous transcription factors. Mol. Cell Biol. 11:267-280.
124. Muroya, S., I. Nakajima, and K. Chikuni. 2002. Related expression of MyoD and Myf5 with myosin heavy chain isoform types in bovine adult skeletal muscles. Zoolog. Sci. 19:755-761.
125. Sartorelli, V., K. A. Webster, and L. Kedes. 1990. Muscle-specific expression of the cardiac a-actin gene requires MyoD1, CArG-box binding factor, and Sp1. Genes Dev. 4:1811-1822.
126. Schiaffino, S. and G. Salviati. 1997. Molecular diversity of myofibrillar proteins: isoforms analysis at the protein and mRNA level. Methods Cell Biol. 52:349-369.
127. Spangenburg, E. E. and F. W. Booth. 2003. Molecular regulation of individual skeletal muscle fibre types. Acta Physiol Scand. 178:413-424.
128. Buckingham, M., L. Bajard, T. Chang, P. Daubas, J. Hadchouel, S. Meilhac, D. Montarras, D. Rocancourt, and F. Relaix. 2003. The formation of skeletal muscle: from somite to limb. J. Anat. 202:59-68.
129. Grobet, L., D. Pirottin, F. Farnir, D. Poncelet, L. J. Royo, B. Brouwers, E. Christians, D. Desmecht, F. Coignoul, R. Kahn, and M. Georges. 2003. Modulating skeletal muscle mass by postnatal, muscle-specific inactivation of the myostatin gene. Genesis. 35:227-238.
130. McPherron, A. C. and S. J. Lee. 1997. Double muscling in cattle due to mutations in the myostatin gene. Proc. Natl. Acad. Sci. U. S. A 94:12457-12461.
131. McPherron, A. C., A. M. Lawler, and S. J. Lee. 1997. Regulation of skeletal muscle mass in mice by a new TGF-beta superfamily member. Nature 387:83-90.
132. Wegner, J., E. Albrecht, I. Fiedler, F. Teuscher, H. J. Papstein, and K. Ender. 2000. Growth- and breed-related changes of muscle fiber characteristics in cattle. J. Anim Sci. 78:1485-1496.
133. Gonzalez-Cadavid, N. F. and S. Bhasin. 2004. Role of myostatin in metabolism. Curr. Opin. Clin. Nutr. Metab Care 7:451-457.
134. Guttridge, D. C. 2004. Signaling pathways weigh in on decisions to make or break skeletal muscle. Curr. Opin. Clin. Nutr. Metab Care 7:443-450.
135. Martin, P. T. 2003. Role of transcription factors in skeletal muscle and the potential for pharmacological manipulation. Curr. Opin. Pharmacol. 3:300-308.
136. Zhao, S. H., D. Nettleton, W. Liu, C. Fitzsimmons, C. W. Ernst, N. E. Raney, and C. K. Tuggle. 2003. Complementary DNA macroarray analyses of differential gene expression in porcine fetal and postnatal muscle. J. Anim Sci. 81:2179-2188.
137. Yao, J., P. M. Coussens, P. Saama, S. Suchyta, and C. W. Ernst. 2002. Generation of expressed sequence tags from a normalized porcine skeletal muscle cDNA library. Anim Biotechnol. 13:211-222.
138. Farber, C. R., N. E. Raney, D. L. Kuhlers, K. Nadarajah, and C. W. Ernst. 2003. Mapping of porcine genetic markers generated by representational difference analysis. Anim Biotechnol. 14:87-102.
139. Schulz, J.S., N. Palmer, J. Steckleberg, S.J. Jones, and M. Zeece. 2006. Microarray profiling of skeletal muscle sarcoplasmic reticulum proteins. Biochim. Biophysic. Acta. 1764:1429-1435.
140. Rilington, V.D., N.E. Raney and C.W. Ernst. 2006. Radiation hybrid mapping of 24 porcine skeletal muscle expressed sequence tags. Anim. Genet. 37:302-304.
141. Xi, G., M. R. Hathaway, W. R. Dayton, and M. E. White. 2007. Growth factor messenger ribonucleic acid expression during differentiation of porcine embryonic myogenic cells. J. Anim Sci. 85:143-150.
142. Taniguchi, M., L. Guan, J. Basarab, M.V. Dodson and S.S. Moore. 2008. Comparative analysis of gene expression profiles in subcutaneous fat tissues of beef cattle. Comparative Biochemistry and Physiology (Part D; Genomics and Proteomics) 3(4):3:251-256
143. Taniguchi, M., L.L. Guan, B. Zhang, M.V. Dodson, E. Okine and S.S. Moore. 2008. Gene expression patterns of bovine perimuscular adipocytes during adipogenesis. Biochemical Biophysical Research Communications 366:346-351
144. Lowell, B. B., N. B. Ruderman, and M. N. Goodman. 1986. Evidence that lysosomes are not involved in the degradation of myofibrillar proteins in rat skeletal muscle. Biochem. J. 234:237-240.
145. Lecker, S. H., V. Solomon, W. E. Mitch, and A. L. Goldberg. 1999. Muscle protein breakdown and the critical role of the ubiquitin-proteasome pathway in normal and disease states. J. Nutr. 129:227S-237S.
146. Furuno, K. and A. L. Goldberg. 1986. The activation of protein degradation in muscle by Ca2+ or muscle injury does not involve a lysosomal mechanism. Biochem. J. 237:859-864.
147. Salem, M., J.Killefer. Cloning of the calpain regulatory subunit cDNA from fish revelas a divergent domain V. Anim. Biotech. 15:1-13, 2004.
148. Saito, M., H. Li, V.F.Thompson, N. Kunisaki, and D.E. Goll. 2007. Purification and characterization of calpain and calpastatin from rainbow trout, Oncorhynchus mykiss. Comp. Biochem. Physiol. 146: 445-455.
149. Goll, D.E., G. Neti, S.W. Mares, and V.F. Thompson. 2008. Myofibrillar protein turnover: the proteasome and the calpains. J. Anim. Sci: 86: E19-E35.
150. Underwood, K.R, W.J. Means, and M. Du. 2008. Caspase 3 is not involved in the postmortem tenderization of beef. Journal of Animal Science, 86: 960-966.
151. Dayton, W. R., D. E. Goll, M. H. Stromer, W. J. Reville, M. G. Zeece, and R. M. Robson. 1975. Some properties of a Ca2+-activated protease that may be involved in myofibrillar protein turnover. In: E. Reich, D. B. Rifkin, and E. Shaw (Eds.) Cold Spring Harbor Conferences on Cell Proliferation, Vol 2, "Proteases and Biological Control". pp. 551-577. Cold Spring Harbor Laboratory Press, Cold Spring Harbor, New York.
152. Kumamoto, T., W. C. Kleese, J. Cong, D. E. Goll, P. R. Pierce, and R. E. Allen. 1992. Localization of the Ca2+ -dependent proteinases and their inhibitor in normal, fasted, and denervated rat skeletal muscle. Anat. Rec. 232:60-77.
153. Badalamente, M. A. and A. Stracher. 2000. Delay of muscle degeneration and necrosis in mdx mice by calpain inhibition. Muscle Nerve 23:106-111.
154. van der Westhuyzen, D. R., K. Matsumoto, and J. D. Etlinger. 1981. Easily releasable myofilaments from skeletal and cardiac muscles maintained in vitro. Role in myofibrillar assembly and turnover. J. Biol. Chem. 256:11791-11797.
155. Peng, I. and D. A. Fischman. 1991. Post-translational incorporation of actin into myofibrils in vitro: evidence for isoform specificity. Cell Motil. Cytoskeleton 20:158-168.
156. Goldfine, S. M., S. Einheber, and D. A. Fischman. 1991. Cell-free incorporation of newly synthesized myosin subunits into thick myofilaments. J. Muscle Res. Cell Motil. 12:161-170.
157. Swartz, D. R. 1999. Exchange of alpha-actinin in isolated rigor myofibrils. J. Muscle Res. Cell Motil. 20:457-467.
158. Lee, H. S., R. M. Bellin, D. L. Walker, B. Patel, P. Powers, H. Liu, B. Garcia-Alvarez, J. M. de Pereda, R. C. Liddington, N. Volkmann, D. Hanein, D. R. Critchley, and R. M. Robson. 2004. Characterization of an actin-binding site within the talin FERM domain. J. Mol. Biol. 343:771-784.
159. Carlsson, L., C. Fischer, G. Sjoberg, R. M. Robson, T. Sejersen, and L. E. Thornell. 2002. Cytoskeletal derangements in hereditary myopathy with a desmin L345P mutation. Acta Neuropathol. (Berl) 104:493-504.
160. Bellin, R. M., T. W. Huiatt, D. R. Critchley, and R. M. Robson. 2001. Synemin may function to directly link muscle cell intermediate filaments to both myofibrillar Z-lines and costameres. J. Biol. Chem. 276:32330-32337.
161. Schweitzer, S. C., M. W. Klymkowsky, R. M. Bellin, R. M. Robson, Y. Capetanaki, and R. M. Evans. 2001. Paranemin and the organization of desmin filament networks. J. Cell Sci. 114:1079-1089.
162. Carlsson, L., Z. L. Li, D. Paulin, M. G. Price, J. Breckler, R. M. Robson, G. Wiche, and L. E. Thornell. 2000. Differences in the distribution of synemin, paranemin, and plectin in skeletal muscles of wild-type and desmin knock-out mice. Histochem. Cell Biol. 114:39-47.
163. Sultana, S., S. W. Sernett, R. M. Bellin, R. M. Robson, and O. Skalli. 2000. Intermediate filament protein synemin is transiently expressed in a subset of astrocytes during development. Glia 30:143-153.
164. Bellin, R. M., S. W. Sernett, B. Becker, W. Ip, T. W. Huiatt, and R. M. Robson. 1999. Molecular characteristics and interactions of the intermediate filament protein synemin. Interactions with alpha-actinin may anchor synemin-containing heterofilaments. J. Biol. Chem. 274:29493-29499.
165. Wang, X., G.H. Hockerman, H.W. Green, C.F. Babbs, S.I. Mohammad, D.E. Gerrard, M.A. Latour, B. London, K.M. Hannon and A.L. Pond. 2006. Merg1a K+ channel induces skeletal muscle atrophy by activating the ubiquitin-proteasome pathway. FASEB J. 20(9):1531-1533.
166. Greaser, M.L., P.R. Krzesinski, C.M. Warren, B. Kirkpatrick, K.S. Campbell, and R. L. Moss. 2005. Developmental changes in rat cardiac titin/connectin: transitions in normal animals and in mutants with a delayed pattern of isoform transition. J. Mus. Res. & Cell Motil 26:325-332.
167. Barash, I., M.-L. Bang, L. Mathew, M. L. Greaser, J. Chen, and R. L. Lieber. 2007. Structural and regulatory roles of the muscle ankyrin repeat protein family in skeletal muscle. Am.J. Physiol. Cell Physiol. 293:C218-C217
168. Davis, J.D., C. Alionte, T. Kobayashi, R.J. Solaro, D.R. Swartz, and S. Tikunova. 2007. Effects of thin and thick filament proteins on calcium binding and exchange with cardiac troponin C. Biophys. J. 92: 3195-3206.
169. Greaser, M.L., C. M. Warren, K. Esbona, W. Guo,Y. Duan, A. M. Parrish, P. R. Krzesinski, H. S. Norman, S. Dunning, D. P. Fitzsimons, and R. L. Moss. 2008. Mutation that dramatically alters rat titin isoform expression and cardiomyocyte passive tension. J. Molec. Cellul. Cardiol. 44:982-991.
170. Kojic, S., E. Medeot, E. Guccione, H. Krmac, I. Zara, V. Martinelli, G. Valle, and G. Faulkner. 2004. The Ankrd2 protein, a link between the sarcomere and the nucleus in skeletal muscle. J. Mol. Biol. 339:313-325.
171. Fernyhough, M.E., Helterline, D.L.,Vierck, J.L., Hausman, G.J., Hill, R.A. and Dodson, M.V. (2005). Dedifferentiation of mature adipocytes to form adipofibroblasts: 1. More than just a possibility Adipocytes 1:17-24.
172. Fernyhough, M.E., G.J. Hausman and M.V. Dodson. 2008. Progeny from dedifferentiated adipocytes display protracted adipogenesis. Cells, Tissues, Organs 188:359-372.
173. Kuang S, Gillespie M, Rudnicki MA. 2008. Niche regulation of muscle satellite cell self-renewal and differentiation. Cell Stem Cell 2: 22-31.
174. Seale P, Bjork B, Yang W, Kajimura S, Chin S, Kuang S, Scime A, Devarakonda S, Conroe H, Erdjument-Bromage H, Tempst P, Rudnicki MA, Beier DR, Spiegelman BM. 2008. PRDM16 Controls a Brown Fat/Skeletal Muscle Switch. Nature, 454:961-7.
175. Fernyhough, M.E., G.J. Hausman, L.L. Guan, E. Okine, S.S. Moore and M.V. Dodson. 2008. Mature adipocytes may be a source of stem cells for tissue engineering. Biochemical Biophysical Research Communications 368(3):455-457.
176. Kuang S, Rudnicki MA. 2008. Emerging biology of satellite cells and their therapeutic potentials. Trends Mol Med 14: 82-91.
177. Witt, C. C., Y. Ono, E. Puschmann, M. McNabb, Y. Wu, M. Gotthardt, S. H. Witt, M. Haak, D. Labeit, C. C. Gregorio, H. Sorimachi, H. Granzier, and S. Labeit. 2004. Induction and myofibrillar targeting of CARP, and suppression of the Nkx2.5 pathway in the MDM mouse with impaired titin-based signaling. J. Mol. Biol. 336:145-154.
177b. Arber, S., Halder,G. and Caroni,P. 1994. Muscle LIM protein, a novel essential regulator of myogenesis, promotes myogenic differentiation. Cell 79:221-231.
177c. Papalouka, V, Arvanitis DA, Vafiadaki E, Mavroidis M, Papadodima SA, Spiliopoulou CA, Kremastinos DT, Kranias EG, Sanoudou D. 2009. Muscle LIM protein interacts with cofilin 2 and regulates F-actin dynamics in cardiac and skeletal muscle. Mol Cell Biol. 29:6046-58.
177d. Linke WA. 2008. Sense and stretchability: The role of titin and titin-associated proteins in myocardial stress-sensing and mechanical dysfunction. Cardiovasc Res. 77:637-48.
178. Mozdziak, P. E., T. J. Walsh, and D. W. McCoy. 2002. The effect of early posthatch nutrition on satellite cell mitotic activity. Poult. Sci. 81:1703-1708.
179. Mozdziak, P. E., J. J. Dibner, and D. W. McCoy. 2002. The effect of early posthatch starvation on calpain mRNA levels. Comp Biochem. Physiol B Biochem. Mol. Biol. 133:221-226.
180. Mozdziak, P. E., J. J. Evans, and D. W. McCoy. 2002. Early posthatch starvation induces myonuclear apoptosis in chickens. J. Nutr. 132:901-903.
181. Pophal, S., J. J. Evans, and P. E. Mozdziak. 2003. Myonuclear apoptosis occurs during early posthatch starvation. Comp Biochem. Physiol B Biochem. Mol. Biol. 135:677-681.